Abstract
To reduce the trend of scouring, understanding the flow pattern around the bridge piers is necessary. By using hydraulic structures such as submerged vanes, it is possible to alter the flow pattern of water, thus scouring process and sediment transport in riverbeds. Since the scouring mechanism for pier groups differs from single pier, experiments were conducted in a 180° sharp bend channel in the laboratory to investigate the flow pattern around pier group and single pier under the influence of 25% submerged vanes. Furthermore, a comparison was made between the flow patterns in single pier and pier group conditions. Three-dimensional velocities along the bend and different depths were measured using an Acoustic Doppler Velocimeter (ADV(. The results suggest that the maximum vorticity value at the apex position of the bend (location of piers) and the maximum secondary flow value, at the distance between the piers and the vanes, were found to be, 0.15 and 0.89, respectively. In the twin pier experiment, the maximum Reynolds shear stress value \({\tau }_{yx}\) decreased by about 36%, and the minimum value decreased by about 49% compared to the single pier experiment. The final results indicated that using submerged vanes obtained the maximum \({\tau }_{yx}\) and \({\tau }_{zx}\) near the vanes. Near the bed and mid-depth of the water flow, the geometric location of the maximum velocity also occurred around the vanes in both experiments. Therefore, the vanes are important in altering the water flow pattern, diverting the flow from around piers and consequently reducing the bridge pier scouring.
Similar content being viewed by others
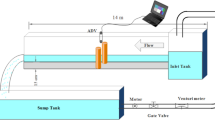
Avoid common mistakes on your manuscript.
Introduction
Well applicable in many instances, such as establishing road connections, bridges represent one of the most salient hydraulic structures. These structures progressively erode in confrontations with natural causes like floods. In addition, the confluence of two streams in rivers also leads to complex water flow behaviour, which, in case of an increase in hydraulic resistance of the main channel, results in turbulent mixing and energy losses that in turn affect the scouring of piers and morphology of rivers bed (Hassan and Shabat 2023). Therefore, to prevent any damage, it is necessary to understand the hydrodynamic behaviour of the river, especially around the bridge piers. Nowadays, consideration of proper measures in hydraulics such as using protective and flow diversion structures such as submerged vanes and recognition of the flow pattern around the piers can help increase the bridge pier’s longevity to some extent.
Odgaard and Wang (1991) considered the submerged vanes’ role in controlling bed sediments in a 90° bend and a straight path. As suggested by their findings, this role involves generating a secondary circulation in the water flow. Turning to the numerical method, Sinha and Marelius (2000) addressed the flow pattern around submerged vanes in a straight channel. The results indicated the generation of a horseshoe vortex on one pressure side of the submerged vane stimulated a motion in the downstream particles alongside the vane and one in the upstream particles alongside the scour hole. Blanckaert and Graf (2001) examined flow patterns and turbulence in a 120° bend channel. They concluded that the maximum velocity took place within the range of the central axis to the vicinity of the outer bank at the 60° cross section. Marelius (2001) undertook two small-scale wave basin experiments in the river with the intention to investigate the performance of submerged vanes in protecting the river bank. The findings were indicative of a reduction in the flow velocity near the bank at the upstream sections of the submerged vanes. As determined by the experimental results of Graf and Istiarto (2002), which was aimed to inspect the flow pattern inside the scour hole about a single pier in a straight channel, a robust vortex system (a horseshoe vortex) was developed upstream of the pier and a flow reversal towards the water surface was generated behind the pier. Tan et al. (2005) administered their experiments under flow pattern investigation around submerged vanes in a straight channel. As claimed in their results, the height of the submerged vanes should be approximately one-fifth (0.2 times) the water flow height for better sediment diversion. The results of the experiments carried out by Han et al. (2011) on the effect installing single and triplet submerged vanes had demonstrated that velocity distribution in a 90° bent channel was steadier than that in the occasion where no submerged vanes were used. Das et al. (2013a) reviewed their experiments by altering varied water flow parameters with the aim of studying the horseshoe vortex system and its circulation on the scour about a single bridge pier inside a straight channel. Their results suggest that streamlines above the initial bed are horizontal and those around the piers are downward. Azizi et al. (2016) carried out a numerical study of the flow pattern around a single pier coupled with submerged vanes at different angles and numbers. According to the results, the test containing 6 vanes placed at the 30° angle demonstrated a better performance than the rest of the tests. Experimental results obtained by Vaghefi et al. (2016); Akbari and Vaghefi (2017), investigating the flow pattern in a 180° bend channel, revealed that the maximum shear stress at bottom layers was transferred from the 40° angle to higher layers at the 60° angle. Additionally, the main vortex’s core was displaced from the inner bank’s vicinity to the middle of the channel from the apex of the bend to the end. Akhtari and Seyedashraf (2018) carried out experimental and numerical investigations to examine the effect of a submerged vane on flow properties within a 60° sharp channel’s curve. As claimed in their results, by installing vanes in the middle of the channel, the secondary flow is weakened. Aminoroayaie Yamini et al. (2018) modelled the scouring around offshore wind turbine using flow 3D software. The modelling results indicated that an increase in the diameter of the pile leads to an increase in scouring. Chooplou and Vaghefi (2019) performed tests with the purpose of scrutinizing the flow pattern around a solitary single pier affected by vanes of 75% submergence ratio in a 180° sharp channel. These tests included examination of the effect of vanes’ displacement at channel width on shear stress and velocity around a single pier. The results demonstrated that the maximum power of the secondary flow occurred in the centre of the channel in the area of the pier and submerged vanes. Experimental investigations administered by Guan et al. (2019) inspected the horseshoe flow around the scour hole of a solitary single pier. As indicated by their results, the power and the magnitude of the main vortex rose with the increase in the scour hole size. Moghanloo et al. (2020) looked into the effect of adding to the collar thickness on an oblong bridge pier’s surrounding flow pattern in a 180° sharp channel. According to their results, the maximum resultant velocity in the first half of bend was towards the inner bank and it shifted orientation in the second half towards the outer bank. Sharma and Ahmad (2020) explored the influence the serial arrangement of submerged vanes could have on flow properties, such as the turbulence kinetic energy, the Reynolds stress, and the flow velocity profile, in a straight channel. They concluded that use of submerged vanes in the channel decreased bed shear stress and bed turbulence compared to the channel with no vanes. Asadollahi et al. (2021) considered the flow and scour patterns around a solitary single pier and a group of triad piers installed transverse to the flow direction. In their work, they compared and analysed the results of the tests in numerical and experimental tests in a 180° sharp bend. They found that SSIIM software performs poorly in simulating the flow pattern of the experiment with a mobile bed, and it provides closer results in simulating experiments with a solid bed. Vaghefi et al. (2021) carried out their numerical research on scour pattern at triad piers installed either longitudinal or transverse to the flow direction by using SSIIM. The experimental findings of a 180° bend were compared with those with relative curvature radii of 2, 3, 4 and 5. The most significant changes in the bed topography occurred in the transverse piers experiments in bend with a relative curvature radius of 2. According to the findings of the modelling by Chauhan et al. (2022) regarding the optimisation of the dimensions of submerged vanes, it was observed that with an increase in the angle of the vanes, the horseshoe vortex extended. Safaripour et al. (2022) experimentally addressed the scouring phenomenon around groups of piers, in a longitudinal direction or transverse to the flow direction, in a 180° sharp bend channel in light of placing vanes of 25, 50 and 75% submergence. Their results indicated that the scouring of the longitudinal pier groups had the lowest scouring rate compared to the rest of experiments. Abdulkathum et al. (2023) analysed the numerical solution of scouring around a single pier with different dimensions under various flow conditions using various prediction models in a rectangular channel. The numerical and experimental modelling results by Tasar et al. (2023) on the impact of placing 6 double-row vanes in an open channel demonstrated that the vanes were capable of reducing flow velocity. Vaghefi et al. (2023) investigated the effect of the number of vanes on the scour depth around a single pier in a 180° sharp bend. The study also revealed that the maximum value of kinetic energy of single pier with two vanes occurred at the location of the pier.
In the study by Safaripour et al. (2022), an investigation of scouring and changes in bed topography (changes in scour depths and sedimentary ridge) and around longitudinal and transvers pier groups under the influence of different submergence ratios of submerged vanes has been addressed. The current research focused on flow patterns, examination of three-dimensional velocities, and data analysis along the bend resulting from the placement of a single pier and a twin pier groups in the presence of submerged vanes. This study presents a completely different subject matter compared to the previous study by Safaripour et al. (2022). The perspective of this study is hydrodynamic, focusing on the analysis of velocity components. The research by Chooplou and Vaghefi (2019) differed from the present research in terms of the number of piers, the submergence ratios of vanes, and the location of vanes within the channel. The current study utilised a twin pier group and a single pier with 25% submerged vanes (close to the water surface). The placement of vanes was in the central axis of the channel, whereas Chooplou and Vaghefi (2019) examined the effect of changing the distance between submerged vanes (deep submergence) from the inner and outer banks on the flow pattern of the single pier.
With respect to hydraulic research around piers, there are a smaller number of studies carried out on flow patterns than scouring analyses because of the challenges involved in carrying out the experiments and the need for advanced facilities and equipment. One of the main challenges in conducting flow pattern tests is maintaining the integrity of the bed and preventing its deterioration over test time. Considering that velocity measurements take about 20 days for each experiment, if there is a bed failure, the test stages need to be repeated. Another issue for data analysis is the need to convert the format of the recorded velocities. Additionally, in some cases, polar velocities must be converted to Cartesian, or vice versa.
As the recent literature on flow pattern suggests, most studies have been carried out in straight channels, leaving only a few to address the topic in bent channels. Because the flow pattern in the bend is more complicated than that in the straight channel due to the presence of transverse flows and their interaction with the longitudinal flow, resulting in the formation of a helical flow. By installing the pier groups in the bend, this complexity is doubled and determining the flow pattern takes time. For this reason, the researchers limited their research to investigating the flow pattern in a bend or a bend combined with a bridge pier. In the investigation of submerged vanes at the upstream side of the pier, limited studies such as research of Chooplou and Vaghefi (2019) have been observed on the flow pattern around a single pier with submerged vanes. Therefore, the flow pattern and the hydrodynamic behaviour of water flow under the influence of pier groups with the upstream submerged vanes located in the bend have not been investigated yet. Therefore, this study focused on examining and comparing the flow pattern around the single pier and the twin pier group under the influence of upstream submerged vanes located in the centre of a channel with a sharp bend. In addition, comparisons have been made to determine the difference between the presence of the single pier and the twin pier group at the downstream side of the submerged vanes, which is the innovation of this research, actually comparing these two states in the changes that occur in various hydrodynamic parameters.
The aim of this research was to determine the flow pattern between the state of establishment of a twin pier group with a single pier in the presence of upstream submerged vanes and to compare the three-dimensional velocities between these two states to investigate the effect of the performance of the upstream submerged vanes with an increasing number of piers on the flow velocity, streamlines, and hydrodynamic parameters. This paper has also undertaken a study of 3D flows’ properties, vorticity, the secondary flow power, the Reynolds stress, etc., comparing them between installations of a single pier and twin piers in a 180° sharp bend with constant flow properties and submerged vanes.
Materials and methods
Flow pattern tests were run in a channel made up of two paths, the upstream one being 6.5 m long and the downstream one being 5.1 m long. A 180° bend with outer and inner curvature radii of, respectively, 2.5 and 1.5 m (with a relative curvature radius of 2) connected these two (Vaghefi et al. 2016). In this study, the bridge piers were tested as a solitary single pier and a group of twin piers transverse to the flow direction. The piers diameter was considered 5 cm. Twin transverse piers were implemented at 4D (D denotes the pier diameter) intervals. The main aim of this study was to explore the flow pattern around the piers. Therefore, 4D was selected as the distance between the piers for locating the ADV in between them. Therefore, the piers caused 10% blockage. The submerged vanes were 7.5 (2.5D) and 1 cm, respectively, at length and thickness according to Safaripour et al. (2022), and they were placed at the horizontal angle of 25 degrees. The distance of the vanes from the pier was considered 5D, according to Abdi Chooplou et al. (2018), and the distance of the vanes from each other was considered 3D (submerged vanes specifications were the fixed parameters of this research).The properties of the piers and the vanes are presented in Table 1. The symbols used in the tests in this table are P as an abbreviation of the pier, T as Transverse, and V as vanes.
This research examines the variations in flow patterns between a twin pier group and a single pier in the presence of upstream submerged vanes. As mentioned, other influential parameters such as the distance of the vanes from the pier, the horizontal angle of the submerged vanes to the flow direction, and the overlap of the submerged vanes have been considered constant in this study, utilising results and past research to determine the values of these parameters.
The discharge in the experiments of this research was determined and fixed using an ultrasonic flow meter (Fig. 1a). This flowmeter had two sensors that were connected to the pump inlet pipe (Fig. 1b). To prevent further vibration by the pump, inlet and outlet pipes had no contact with the laboratory channel. The way these sensors worked was that sound waves were sent into the tube by the first sensor, and the second sensor received these waves and calculated the speed of the passage with an accuracy of ± 1 mm/s. Then, by entering the diameter of the pipe and the distance between the sensors, the flow rate was obtained by the device. Therefore, the discharge of 70 L per second and the water depth of approximately 18 cm were considered throughout the tests.
The flow was maintained under clear water conditions. Scour tests were carried out under incipient motion conditions as per the Froude number of 0.29, the Reynolds number of approximately 51,400 and \(\frac{u}{{u}_{c}}\)=0.98 (the ratio of velocity to critical velocity). The flow steady was considered in the bend, and the flow was uniform at the upstream straight path of the bend. The channel bed material was coated using sediments with an average grading diameter of 1.5 mm and a geometric standard deviation of 1.14. This is because according to the results of Raudkivi and Ettema (1983), the average diameter of particles should be larger than 0.7 to prevent rippling, and based on the findings of Chiew and Melville (1987), the standard deviation should be less than 1.3.
Implementation of the piers and the vanes in flow pattern tests is depicted in Fig. 2.
Vectrino 3D velocimeter, called ADV, was utilised for measuring the flow velocity’s 3D components. The user can adjust the velocity on this device within the range of ± 0.01 to ± 7 m. In this work, the velocity ranged from ± 0.01 to ± 1 m/s, and the device frequency was 25 Hz (Akbari et al. 2021). Assuming a duration of 1 min, up to a maximum of 1521 flow velocity data were collected in three directions and at every node of the established mesh grid for each second. Needless to say, the collection time at each node increased to 2 min at points near the pier or the submerged vanes. This device had one side-looking and one down-looking probe. This device collects velocity data by using each probe at a different water depth. These receivers receive the waves sent by the sensor. The down-looking and side-looking probes record the water velocity within a range of 5 cm, respectively, lower and before the location of the receivers. Hence, the down-looking probe cannot be employed to record velocity at the water surface level. With the help of the side-looking probe, the flow velocity could be measured at areas in proximity of the channel walls and the water surface.
Figure 3 depicts the process of installing the velocimetry device at the 180° bend and the relevant hardware for data storage. In this figure, a laptop is connected to the body of the velocimeter with a cable. The probe shown in this figure is side-looking, which was adjusted for collecting velocity data in regions near the channel wall at the water surface level.
As velocity collections were carried out with the aim of determining the flow pattern around the structures (piers and vanes) and the variations in the flow occurred along the bend, then they had to be done in the channel in accordance with bed topography variations with proper meshing. Therefore, the velocities in the vicinity of the piers and the vanes were collected at short intervals. Moreover, along the channel, due to bed topographic changes downstream of the piers and generation of the secondary scour hole, velocity collection was undertaken with a fine mesh in these regions. Figure 4 displays the mesh required for these tests as an instance. This mesh is divided into 50 longitudinal sections. The sections around the piers and vanes were selected at short longitudinal and transverse intervals for velocity measurements. The velocities across the sections in proximity of the piers and the vanes as well as those at the end of the bend were collected at cross sections at 2-cm intervals (50 cross sections) due to occurrence of significant bed topography variations, and those across the rest of the bend were collected at 5-cm intervals (20 cross sections). In addition, 6 levels (+ 1, + 3, + 6, + 10, + 14, and + 17 cm) were considered at channel depth, and as for the regions with bed undergoing scour, the velocities were collected at levels lower than the initial bed level (− 2, − 4, − 6, and − 8 cm) per the scour depth value.
Description of the experiments
There are definitely differences between a laboratory model and a real river, using real models is often very complex and sometimes unfeasible. For instance, Fig. 5 demonstrate these differences. As can be seen in Fig. 5a, the erosion of the river bed and banks constantly changes the water flow pattern and the morphology of the river bed. In the laboratory, the walls are rigid and non-erodible. Conducting experiments under river conditions where u/uc varies is completely different. In this study, initially, scouring experiment was conducted close to mobile bed conditions in the upstream straight path (\(\frac{u}{{u}_{c}}\)= 0.98). As it may have been noticed, the highest amount of scouring and changes in bed topography occurred in this case. After the equilibrium of the bed was realized and the bed was fixed with a specific adhesive, the flow pattern test stages were carried out. In addition, the relative curvature radius in the laboratory channel is constant, whereas, as seen in Fig. 5b, the relative curvature radius in some rivers’ bends is not constant. These are issues that cause the laboratory conditions to differ from the real river.
Flow pattern experiments were carried out after the bed topography variations reached equilibrium and the bed was made rigid so that after a 9-h equilibrium time (the relative equilibrium time), the pump was gradually turned off, and after full bed drainage and the drying of the bed materials, its surface across the channel was covered with a thin layer of concrete adhesive using an air compressor because fixing the materials required high precision, and bed topography was not to undergo any changes. In addition, because recording the velocities at longitudinal, transverse, and vertical directions required a great amount of time in flow pattern tests, the pump had to work for a long time. Therefore, after the concrete adhesive dried out, the bed was fixed again using fibreglass adhesive to assure that no failures were found during the flow pattern test. Figure 6 illustrates the fixed topography in the 2PT-2V25 test (a) around the piers and (b) along the downstream path after installation of the piers a few days following the bed surface’s drying out and being fixed. The flow began with a discharge of 70 L per second so that the test could be performed, and the velocities were collected by ADV as per the intended mesh grid. The results of velocity collection, the flow pattern parameters determination and their discussion are provided in the following sections.
Results and discussion
As depicted in Figs. 7, 8 and 9, woodchips and coloured ribbons were, respectively, used on water surface and at depth to demonstrate the qualitative flow pattern about piers and vanes. An attempt has been made in Fig. 7 to illustrate how wake vortices are developed behind the bridge pier at the water surface level through 4 phases. Pouring the woodchips behind the bridge pier, as shown in Fig. 7a, made a clockwise vortex appear in that area. As shown in Fig. 7b, the clockwise vortex gradually approached the outer bank, and a counterclockwise vortex was developed on the other side of the pier. These vortices were generated owing to the flow separation phenomenon and shear layer formations. As it is also evident in Fig. 7c and d, vortices were developed horizontally and were rotating around the vertical axis. As for Fig. 7c and d, the vortices distanced away from each other, and the clockwise vortex was smaller than the counterclockwise vortex; this wake vortex generated at the water surface was deviated towards the outer bank.
Display of the wake vortices created behind the bridge pier by woodchips in 2PT-2V25 experiment a Formation of the first clockwise wake vortex; b formation of the second counterclockwise wake vortex; c clockwise wake vortex inclining towards the outer bank; and d counterclockwise wake vortex inclining towards the inner bank
A view of the qualitative flow pattern a diversion of the streamlines towards the outer bank (water surface level) in P-2V25 experiment; b the streamlines at layers near the bed and water surface in P-2V25 experiment; c the inner bank in the downstream half of the bend at the water surface level in P-2V25 experiment; and d upstream of the submerged vanes in 2PT-2V25 experiment
Moreover, in consecutive pictures, Fig. 8 displays the phases through which vortices are formed downstream of the vanes. As observed in this figure, green and yellow ribbons downstream of the vane depict the phases of development for counterclockwise vortices. The onset of the development of vertical vortices is visible in Fig. 8a. At the beginning, the vortices were smaller in size (Fig. 8b). In every part of Fig. 8, illustration of the streamlines at mid-depth by blue and red ribbons indicates that they interfered with each other.
Further, in Fig. 9a on the qualitative flow pattern, coloured ribbons indicate that the streamlines at the water surface level were deviated towards the outer bank, and in Fig. 9b on the qualitative flow pattern at levels near the initial bed level, due to intensification of the longitudinal pressure gradient, these streamlines (red and blue ribbons) oriented towards the inner bank. With an increase in the flow depth from the bed and a reduction in the longitudinal gradient, the streamlines (yellow and green ribbons) oriented towards the outer bank. It was predicted that an increase in depth entailed the dominance of the secondary flows over the longitudinal pressure gradient, leading to diversion of the streamlines towards the outer bank.
Figure 9c illustrates the qualitative flow pattern in the vicinity of the inner bank in the downstream half of the bend at the water surface level. The blue and red ribbons in this figure follow the flow direction, and the yellow and green ribbons are in line with the water flow as for the part where they fall on the water surface; however, as specified in Fig. 9c, the ends of yellow and green ribbons were influenced by the flow turbulence and were dragged down to the lower layers from the water surface. As specified (area A), these lines at these layers are directed downward. As maintained by this figure, the streamlines at the water surface were in line with the bend because the centrifugal force had overcome the rest of the forces. Figure 9d depicts the streamlines upstream of the submerged vanes. According to this qualitative flow pattern, the streamlines at layers near the bed were diverted towards the inner bank. The angle of inclination in this figure is denoted by Ɵ. In addition, these lines were highly orderly upstream of the submerged vanes (area B), yet the ribbons demonstrated disorder upon approaching the vanes while they were being influenced by the flows around the vanes.
3D flow velocity distribution at certain plan sections
Figure 10 illustrates distribution of tangential (UƟ), radial (Vr) and vertical (Wz) velocities at the level of 5% of the flow depth at the bend entrance from the initial bed level in P-2V25 and 2PT-2V25 tests. Because of proximity to the initial bed level and due to sediment generation downstream, the velocity values at this level were zero near the inner bank. It can be observed in Fig. 10a that the maximum tangential velocity in the bend upstream half occurred in proximity of the inner bank and that in the downstream half took place near the outer bank. At the channel centre, the flow was influenced by the piers and the tangential velocities were lowered. The maximum tangential velocities are almost the same values in both tests. Given the magnified illustration of Fig. 10a, tangential velocity values in both tests are approximately equal up to the position of the piers. Velocity reductions at each cross section downstream of the piers and near the central axis of the bend occurred twice in 2PT-2V25 and once in P-2V25. Such a decline in velocity can be attributed to the effect of the piers and their number (area A).
It can be observed in Fig. 10b that at the level of 1 cm from the initial bed level in the bend’s upstream half, the radial velocities were oriented in the direction of the inner bank and therefore caused lateral streams and scouring in the bend. Therefore, the sediments from this region moved towards the inner bank and developed a sedimentary pile. As shown in this figure, with the twin piers test, the radial velocity values (directed towards the inner bank) increased at the near-bed level in comparison with that of the single pier. Considering the pier’s location and the flow complexity around them, the radial velocity values display fluctuations. Regarding the bend’s downstream half, the radial velocities in P-2V25 near the outer bank (from the central axis to the outer bank) were directed towards the outer bank, but those in 2PT-2V25 were not only directed towards the outer bank but also had greater values. Analysis of the radial velocity values in both tests revealed that the difference of the maximum radial velocity with outer bank orientation was less than 1 cm/s, but the difference of its maximum value with inner bank orientation was approximately 12 cm/s. As observed in the magnified illustration of Fig. 10b, the radial velocity values (directed towards the inner bank) around the pier increased in the twin piers test compared to those in the single pier test.
Figure 10c depicts vertical velocity values (Wz). In this figure, the vertical velocities around the pier showed many fluctuations, and at the downstream side of the pier, their maximum value gradually moved towards the inner bank upon approaching the bend’s ending sections. Regarding its magnified illustration, the vertical velocity values in P-2V25 and 2PT-2V25 were similar from the bend’s central axis to proximity of the outer bank, and the major difference in vertical velocities in these tests was observed at the distance between mid-channel and the inner bank. The maximum vertical velocity, directed towards the flow level, occurred in P-2V25 at a shorter distance from the inner bank than that in 2PT-2V25. The vertical velocities towards the bed happened around the submerged vanes, and the maximum velocity was observed in P-2V25. The difference between vertical velocities directed towards the bed in P-2V25 and 2PT-2V25 tests is around 10%. The maximum value (towards the flow level) in P-2V25 occurred in front of the bridge pier and that in 2PT-2V25 happened at the downstream side of the piers at a distance of approximately 5D from the pier’s vicinity. The maximum vertical velocity in the flow level direction in the single pier test increased by a factor of approximately 4.5 compared to that in the twin piers test.
Figure 11 shows the distribution of tangential and radial velocities at the water surface. When the velocities were collected at the water surface at this level, the vertical velocities were considered zero because the side-looking probe was not fully submerged.
As shown in Fig. 11a, the tangential velocity variations in 2PT-2V25 at the water surface underwent insignificant fluctuations except around the pier, but those in P-2V25 began experiencing fluctuations from approximately the upstream side of the vanes which lasted to approximately 120 degrees. At this level, the flow is sometimes oriented upwards at a negative value. The maximum tangential velocity value opposite to the mainstream’s direction occurred in P-2V25 at a value approximately 1.5 times that in 2PT-2V25. Given Fig. 11a, the maximum tangential velocity in 2PT-2V25 was observed at approximately 67 cm/s near the outer bank at a distance of nearly 35D from the channel’s centre in downstream direction (area A). The tangential velocity variations through the ending sections of the bend were almost the same in both tests. In the downstream half of the bend in both tests, the tangential velocity values decreased near the inner bank. It could be concluded that the water surface flow was not influenced by the piers at the channel’s downstream side. As the magnified illustration of Fig. 11a signifies, where the flow was upward oriented and negative, the tangential velocity values around the piers and vanes increased in P-2V25 in comparison with those in 2PT-2V25. Comparing Figs. 10a and 11a reveals that the maximum tangential velocity values in the bend’s downstream half were almost equal in P-2V25 and 2PT-2V25 tests.
The radial velocities at the level of 5% of the flow depth from the water surface are shown in Fig. 11b. This figure demonstrates that the radial velocity values in the twin piers test decreased compared to the single pier test with orientation towards the outer bank (except for the areas around the piers). The maximum radial velocity with inner bank orientation happened at 90 degrees in the channel, and its value in 2PT-2V25 was almost 1.6 times that in P-2V25. The maximum radial velocity values with orientation towards the outer bank occurred at the interval between the piers and the vanes in 2PT-2V25 and at the downstream side of the pier in P-2V25. The radial velocity values in the downstream half of the bend in 2PT-2V25 declined closer to the outer bank. With regards to this figure, from approximately the 120° angle in 2PT-2V25, the radial velocity values increased within the range of the channel’s central axis to the inner bank in comparison to the outer bank velocities.
An analogy of the flow patterns in plan in Figs. 10 and 11 reveals that the velocity variations grew steadier closer to the water surface; hence, the flow near the bed experienced further turbulence. A comparison made between different distributions of 3D flow velocity components in plan, as shown in Fig. 10, could lead to the conclusion that the maximum turbulence intensity occurred at the site of the piers and the vanes. The results of the present work are comparable to those reached by Chooplou and Vaghefi (2019), which was designed to explore turbulence intensity at the level near the bed, with the maximum longitudinal, transverse, and vertical values happening at the central position of the bend. Moreover, the results of their studies reported the occurrence of transverse turbulence intensity throughout the bend, where the vertical turbulence was less intensified than the longitudinal and transverse ones. Therefore, with a comparison of the transverse velocity distributions in plan in this study, the conclusion could be that the increase in the radial velocities in the downstream half of the bend in 2PT-2V25 in proportion to those in P-2V25 means more intense turbulence. In turn, the secondary flow power in 2PT-2V25 must be greater than that in P-2V25 according to Chooplou and Vaghefi (2019). By examining the distribution of velocities in three directions at the near-bed level and considering the results of their studies, it could be concluded that the minimum turbulence occurred in vertical direction and the maximum turbulence occurred in longitudinal and transverse directions.
3D flow velocity distribution at cross sections
The analogy between 3D tangential, radial, and vertical velocities at cross sections of the pier location in P-2V25 and 2PT-2V25 is shown in Fig. 12. The figures on the left depict the tangential velocities all over the channel width and the figures on the right show the magnified illustration of the range of 34 to 66% of the channel width from the inner bank.
The tangential velocities at the site of the piers in P-2V25 and 2PT-2V25 are compared in Fig. 12a. In this figure, the tangential velocity values are almost equal within approximately the beginning and the ending 30% ranges of the channel width in both tests. The figure suggests that the tangential velocities in P-2V25 decreased from the level of 55% of the flow depth to the water surface (area A). In both tests, the maximum tangential velocity values took place at the level of 14 cm (equivalent to 77% the flow depth at the bend entrance from the initial bed level) and in proximity of the outer bank. The difference between the maximum tangential velocity values in these two tests is about 10%, and the maximum tangential velocity increased by increasing the number of piers (from one to two piers).
Figure 12b depicts the radial velocities in both tests at the cross sections of the pier location. According to this figure, the maximum radial velocity values took place near the inner bank towards inner bank in 2PT-2V25. Given the magnified illustration in Fig. 12b, the radial velocity was directed towards the inner bank at mid-channel up to approximately 30% of the flow depth from the initial bed level, and such an interference between the flow and the longitudinal flow entailed development of lateral flows and the resulting scour (area B). The maximum radial velocity towards the outer bank in both tests took place at the level of 10 cm (equivalent to 55% of the flow depth at the bend entrance from the initial bed level) within the range of 58 to 68% of the channel width from the inner bank, which increased by approximately 48% in the single pier test compared to that in the twin piers test. The maximum radial velocity with orientation towards the inner bank also happened within the range of 24 to 32% of the channel width from the inner bank at the level of − 8 cm in both tests, with a 25% increase in 2PT-2V25 compared to P-2V25. Therefore, the width of the scour around the piers in the twin pier group test was greater than that in the single pier test.
Figure 12c presents the vertical velocities at the pier’s location in P-2V25 and 2PT-2V25. As shown in this figure, the vertical velocity was directed towards the bed at most points. The maximum vertical velocity towards the bed at the level of 14 cm occurred in 2PT-2V25 at the position of 92% of the channel width from the inner bank at approximately 24 cm/s. It can be observed in Fig. 12c that the vertical velocity values in P-2V25 towards the bed increased from the inner bank towards the pier, and the maximum velocity was realized in this direction at a distance of 58% of the channel width from the inner bank at an approximate value of 23 cm/s. In this test, with a gradual departure from the pier’s location, the vertical velocity values (oriented towards the bed) declined up to proximity of the outer bank. The vertical velocity values (oriented towards the bed) underwent a decrease from the inner bank towards the outer bank in 2PT-2V25. Considering the magnified illustration of Fig. 12c, in both tests, the vertical velocity values oriented towards the bed (at lower levels) were greater towards the inner bank than those towards the outer bank.
To analyze 3D velocities and the flow pattern at the site of the vanes, the cross section at a distance of 5D from the channel’s centre in upstream direction (the distance of the vanes from the pier according to Abdi Chooplou et al. 2018) is provided in Fig. 13.
Figure 13a illustrates the tangential velocity distributions at the site of the submerged vanes in both P-2V25 and 2PT-2V25. The tangential velocity values in both tests, except around the vanes, are similar in this figure. This could suggest that the flow reversal in front of the bridge pier also affects the flows around the vane. The tangential velocities at the water surface level underwent more reduction at the gaps between the vanes in P-2V25 than those in 2PT-2V25 (area A). The maximum tangential velocity in the direction of the mainstream happened in P-2V25 at approximately 47 cm/s at the level of 14 cm at a distance of 48% of the channel width from the inner bank. The maximum tangential velocity in the opposite direction to the mainstream’s and with an upward orientation in P-2V25 occurred at the water surface level at approximately 52 cm/s.
With a comparison of the radial velocities in both tests in Fig. 13b, it can be observed that the radial velocities near the scour hole bed are oriented towards the inner bank, and the combination of this velocity with the tangential velocity entails creation of a scour hole. The maximum radial velocity value in 2PT-2V25 with an inner bank orientation occurred at approximately 20 cm/s at a distance of nearly 38% of the channel width from the inner bank in the vicinity of Vi (the vane close to the inner bank). According to this figure, the radial velocities (with inner bank orientation) have greater values in 2PT-2V25 than those in P-2V25. However, these differences in radial velocities are almost close to each other, and therefore the topographical changes around the vanes in both tests are almost the same.
Figure 13c presents the vertical velocities of both tests. It is observed in this figure that within approximately the beginning and the ending 30% ranges of the channel width, the vertical velocity values in both tests had little difference except at the depth of 14 cm. More disorder in vertical velocity values is observed around the vanes in P-2V25. For instance, the values of such disorder at a distance of 56% of the channel width from the inner bank are evident in Fig. 13c. The maximum velocity (towards the bed) was found in P-2V25 at the level of 14 cm higher than the initial bed level at approximately 57 cm/s. The vertical velocity values (oriented towards the bed) at the gap between the vanes in both tests decreased at the levels near the water surface compared to inner and outer banks’ velocities and increased at the levels lower than the initial bed level.
Comparing the vertical velocities in the vicinity of the pier (Fig. 12c) to those around the vanes (Fig. 13c) suggests that the vertical velocities at cross section at the site of the vanes had many fluctuations in comparison to those at the pier’s location.
As shown in Figs. 12a and 13a, the tangential velocity values were almost the same at the site of the piers and submerged vanes within the approximate range of 30% at the beginning and the ending regions of the channel width. In addition, this result was also observed by Moghanloo et al. (2020); although the bridge pier they used was an oblong pier, similar results were concluded. The flow pattern in this range of the channel width is independent of any structures installed at the central position of the 180° channel. It can be observed in Figs. 12c and 13c that the vertical velocities near the inner bank are oriented towards the flow level, and they shift directions towards the bed upon approaching the outer bank. The same finding was also reported by Moghanloo et al. (2020).
3D flow velocity distribution at longitudinal sections
Distribution of tangential (UƟ), radial (Vr), and vertical (Wz) velocities at longitudinal sections at a distance equal to 50% the channel width from the inner bank in P-2V25 and 2PT-2V25 are illustrated in Fig. 14. At this section, the velocities from the site of the vanes to 7D from the channel’s centre at the downstream side were compared in P-2V25 and 2PT-2V25 at varied levels from the bed. The horizontal axis of the diagram is given per the position at the bend (in degrees) and the vertical axis represents the water depth at different velocity collection levels (in cm).
The tangential velocities in P-2V25 and 2PT-2V25 are compared at the same longitudinal section in Fig. 14a. At 91 degrees, representing the rear area of the bridge pier in P-2V25, the tangential velocities against the mainstream are indicative of return flows, whose continuation is evident at 92.5 degrees at the water surface level and the level of 77% of the flow depth from the initial bed level. The tangential velocity values in 2PT-2V25 at the upstream side of the pier were the same at most points as those in P-2V25; however, at the downstream side of the pier, within the range of 89.5 to 95.5 degrees, the tangential velocity values (with a downward orientation) were greater in 2PT-2V25 than those in P-2V25.
The radial velocity values along mid-channel are shown in Fig. 14b. This figure illustrates the disorderly pattern of the radial velocities. At any angle of either test, the radial velocity values vary across different levels. In general, at the levels near the water surface, the velocities were directed towards the outer bank, and at negative levels, they were directed towards the inner bank. For every level away from the bed, the radial velocity values decreased. Hence, as per the results of Chooplou and Vaghefi (2019), it can be concluded that the turbulence intensity subsided at the levels near the water surface as compared to those near the bed. As specified in the figure (area A), the radial velocity values (oriented towards the outer bank) in both tests declined upstream of the vanes.
As shown in Fig. 14b, at most levels and longitudinal sections, the radial velocity values (oriented towards the inner bank) were smaller in P-2V25 than those in 2PT-2V25. However, from the scour hole bed to the level of 1 cm of the initial bed level within the range of approximately 92.5 degrees to the pier’s downstream side (area B) in P-2V25, the radial velocity values (with inner bank orientation) rose in comparison to those in 2PT-2V25, whereas 2PT-2V25 had the greatest radial velocity values (with inner bank orientation) from the bed to the level of 55% of the flow depth at the bend entrance from the initial bed level within the range of approximately 85 degrees to the pier’s downstream side.
Vertical velocity values at the channel’s central axis in P-2V25 and 2PT-2V25 are provided in Fig. 14c. Considering the comparison between vertical velocities in both tests and their nearly same values, the vertical velocity values were observed to be independent from the arrangement and the number of piers from approximately 94 degrees to the downstream side. According to this figure, the vertical velocity values (oriented towards the bed) were declining from the upstream sections to the downstream side in both tests so that the maximum velocity in this direction came about at the level of 14 cm of the initial bed level, equal to 77% of the flow depth at the bend entrance, within the range of 80.5 to 82 degrees.
Dispersion of tangential (UƟ), radial (Vr), and vertical (Wz) velocities
Figure 15 compares the dispersion of tangential, radial, and vertical velocities in P-2V25 and 2PT-2V25 tests. In every three diagram, the horizontal velocity axis corresponds to every point in 2PT-2V25 and the vertical axis corresponds to the points in P-2V25. It is worth noting that at some points in one test, the velocity was zero, and at the corresponding point in another test, it had values. These points were not considered a part of any region in calculation of the percentage of the points present in regions.
As observed in Fig. 15a, the majority of tangential velocities in both tests have fallen in the first region with almost the same range of variations. According to this figure, about 97.7% of the points appeared in the first region. Very little dispersion is found in regions 2 and 4. At some points, negativity of the tangential velocity is indicative that the flow is negative and shifts upwards, in the opposite direction to the mainstream. These points occurred around the vanes and the piers. About 0.69 and 1.8% of the points, respectively, appeared in regions 2 and 4. Figure 15b illustrates the radial velocity variation range, where most velocities occurred in regions 1 and 2 at respective values of approximately 37.5 and 47.2%. The radial velocity values in regions 3 and 4, respectively, about 3.8 and 11.7%, are smaller than those in the two mentioned regions. The positive radial velocity values in the first region indicate that the velocities were oriented towards the outer bank. Figure 15c illustrates the vertical velocity values in every region. The positive values of the vertical velocities (oriented towards the flow level) in both tests were much smaller than the tangential and radial velocities in the first region so that approximately 14.7% of the vertical velocity values in both tests were positive. Moreover, the vertical velocity values were the greatest, about 43.6%, in region 3. In this region, the vertical velocities in both tests had negative values and the flow was in fact a down flow. Moreover, vertical velocity values in regions 2 and 4 were, respectively, 12.3 and 29.4%.
Table 2 presents the percentage of positive and negative values of 3D velocity components in both tests. As presented in this table, the positive values of the longitudinal velocity values in both tests had the highest values compared to the positive values of the radial and vertical velocities. At most longitudinal, transverse and vertical sections, the longitudinal velocity component had positive values, and the vertical velocity component had negative values.
Analysis of vorticity and secondary flow power
The secondary flow power is a key factor involved in bed scour development as the interaction of the secondary flow with the longitudinal flow means generation of a helical flow, which displaces sediments by interfering with the straight flow of water and shifting it at channel width.
There are numerous factors helping with understanding the secondary flow power, among which vorticity is given as Eq. (1) (Vaghefi et al. 2017). Given the vorticity formula, its value is found zero in straight and non-rotating flows. Vorticity, or the curl of velocity, is in fact the same as rotation of a fluid element of water for ∂x*∂y. It can be calculated for each cell from the velocity collection mesh grid using Eq. (2). The amount of rotation at the cross section and around the longitudinal line is obtained through Eq. (2), which is calculated for each and every cell at the section and is then averaged. Where \({w}_{z}=\) vorticity about the z-axis, u = lateral component of velocity, and v = vertical component of velocity.
The second factor involved in determining the secondary flow power is found using Eq. (3). This equation is the ratio of the lateral kinetic energy to the main kinetic energy (Shukry 1950). In Eq. (3), U, V, and W parameters denote the velocity values on x, y, and z axes, and g represents acceleration of gravity. Where \({V}_{xy}\)= resultant velocity in longitudinal and transverse directions, and \( V\) = resultant velocity in three directions.
Figure 16a presents calculation of vorticity at each angle according to Eq. (2).
Careful examination of Fig. 16a reveals that the vorticity value in both tests increased from the bend entrance. Its value in P-2V25 reduced at the site of the vanes and then rose until it reached maximum at the pier’s location. The value plummeted after the pier’s location to approximately 100 degrees. Then the vorticity values showed little difference and had almost steady variations to the ending sections of the bend. In 2PT-2V25, the variations were on a rising slope up to 40 degrees, but its value underwent a sharp decline there and continued in a sinuous trend to the vicinity of the vanes with little variation. It can be observed in this test that the vorticity value reached maximum at the centre of the channel (the piers’ location). The greatest impact of vorticity in 2PT-2V25 was evident in the bend’s upstream half. The maximum vorticities in both tests were almost similar, and occurred at the same location (the piers’ location). With a comparison between the vorticity value in the downstream half in both tests, it can be concluded that lateral vortices decreased in these regions in 2PT-2V25 compared to those in P-2V25.
The secondary flow power of both tests is shown in Fig. 16b. The secondary flow is further empowered with the water flowing in the bend entrance. In both tests, the maximum secondary flow power values were found within the approximate range of 80.5 to 91 degrees. The reason for the increase in secondary flow strength in this area is the narrowing of the cross section, the increase in the radial velocity component and the tendency to create a region of separated flow. The maximum secondary flow power had occurred within the distance between the vanes and the pier in tests performed by Chooplou and Vaghefi (2019) as well. In 2PT-2V25, its value was on a rise closer to the end of the bend within the approximate range of 130 to 155 degrees. Thereafter, it began to decrease. In P-2V25, it increased from around 140 degrees to the ending sections of the bend, except for 160 degrees. This could be attributed to the impact of the downstream straight path and bed topography variations in addition to existence of the secondary flow. The maximum secondary flow power value occurred in the twin piers group transverse to the flow direction accompanied by vanes for approximately 15.45% at a distance of about 63D from the bend entrance. In the test on the single pier together with the vanes, the maximum secondary flow power value also happened at the same place for approximately 15.13%.
Reynolds stresses
After 3D velocity collections were done by using ADV, velocity fluctuations in various directions were obtained by applying the relevant equations and ExploreV. For example, the products of velocity in three directions were calculated as described below (White 1986).
In Eqs. (4) to (6), u', v', and w' represent fluctuating velocities in three directions (length, width, and depth), and u, v, and w are the mean flow velocities in the same directions.\(\overline{u }\), \(\overline{v }\) and \(\overline{w }\) are, respectively, the average flow velocities in the longitudinal, transverse and deep directions. Using the products of velocity fluctuations obtained from the aforementioned equations, the Reynolds stresses could also be calculated. This only requires consideration of the Reynolds stresses’ direction as the opposite of velocity distributions. Equations (7) to (9) provide the means to calculate the Reynolds stresses. In the following equation, ρ is water density, and the related stresses are the Reynolds stresses (Das et al. 2013b). Where \({\tau }_{zx}\) = shear stress in z coordinate in x direction, \({\tau }_{yx}\) =shear stress in y coordinate in x direction, and \({\tau }_{zy}\) = shear stress in z coordinate in y direction. These stresses are obtained based on fluctuating velocity values.
The Reynolds stresses distributions at the near-bed level, equal to 1 cm above the initial bed level, in P-2V25 and 2PT-2V25 in three directions are illustrated in Fig. 17.
Figure 17a illustrates the distribution of the Reynolds stress, \({\tau }_{yx}\). As for Fig. 17a, the minimum fluctuations in longitudinal and lateral velocities in P-2V25 occurred at the bend’s centre and the site of the vanes, where the Reynolds stress was positive, referring to a powerful secondary flow and negative values of lateral velocity in this region. Given the magnified illustration in the figure, the minimum Reynolds stress values of \({\tau }_{yx}\) at this section are found behind the bridge pier. It can be observed in Fig. 17a in 2PT-2V25 that the minimum velocity occurred around the vanes, and the maximum velocity occurred behind the bridge piers, as it was the case with the solitary single pier. By adding to the number of piers (single to twin), the maximum Reynolds stress of \({\tau }_{yx}\) increased by nearly 36% and its minimum by approximately 49% in proportion to those in the single pier test. Moreover, the minimum Reynolds stress values of \({\tau }_{yx}\) in 2PT-2V25 were noted within the range of 60 degrees in the vicinity of the bend’s ending section.
The distributions of the Reynolds stress, \({\tau }_{zx}\), in P-2V25 and 2PT-2V25 tests are depicted in Fig. 17b. As shown in Fig. 17b, in P-2V25, the maximum value of \({\tau }_{zx}\) decreased by approximately 61% compared to \({\tau }_{yx}\). As for this figure, the maximum \({\tau }_{zx}\) value was realized around Vi (the vane near the inner bank). According to Fig. 17b, the distribution of the Reynolds stress, \({\tau }_{zx}\), in 2PT-2V25 shows more variation than that in P-2V25 so that in the upstream half of the bend, from around 60 degrees to proximity of the vanes, the smallest values of these velocities occurred, the minimum of which appeared within the range of the mid-channel axis to the outer bank’s vicinity. Further, the products of longitudinal and vertical velocities also decreased at the bend outlet from about 170 degrees. The minimum values of \({\tau }_{zx}\) in 2PT-2V25 occurred at a distance of 10% of the channel width from the inner bank at a distance of about 7D from the piers’ location (equal to 100 degrees of the bend).
Figure 17c shows the distribution of the Reynolds stress,\({\tau }_{zy}\). In a comparison between Fig. 17c and Fig. 17a–c reduction is observed in the maximum and minimum values of \({\tau }_{zy}\) compared to \(\tau_{zx}\) and \(\tau_{yx}\) According to Fig. 17c, in P-2V25, most positive points of lateral and vertical velocities’ products appeared in the downstream half of the bend, and their maximum occurred near the inner bank within the approximate range of 103 to 160 degrees. The minimum values also occurred behind the bridge pier at approximately 37 (cm/s)2. In the twin piers test, the minimum value of \({\tau }_{zy}\) decreased by about 63% in comparison to that in the single pier test. On the report of this figure, the minimum and maximum products of lateral and vertical velocities were found around the Pi pier.
For a better understanding of Reynolds stress values in Fig. 17 and due to the large amount of data throughout the channel, the average stress at each cross section is shown in Table 3. According to this table, the average stress in both experiments has been similar from the beginning of the channel to around a 40° angle, indicating that in these areas, the flow pattern is independent of the influence of piers and submerged vanes. Therefore, the scouring of these areas has been completely similar. As Table 3 illustrates, the values of \(\overline{{\tau_{zx} }}\) in the twin pier group experiment have been higher than those in the single pier experiment. The negativity of \(\overline{{\tau_{yx} }}\) from around a 98.5° angle of the bend to the end of the bend in the single pier experiment indicates the scouring of the bed in these areas. However, these values have been positive in the twin pier group experiment. This reveals insignificant changes in the bed topography in this experiment.
Geometric location of maximum resultant velocity along the 180° sharp bend
The results obtained through velocity collections along the channel at every level indicated that some points experienced the maximum possible velocity. For instance, Fig. 18 illustrates the maximum resultant velocity path along the bend at three different levels of 5, 55, and 95% of the flow depth at the bend entrance from the initial bed level.
As for Fig. 18a, it can be observed that in both tests, the geometric location of the maximum velocity in the upstream half of the bend is near the inner bank due to generation of a positive longitudinal pressure gradient. However, given the vanes’ location, the maximum velocity path shifted in its orientation towards the outer bank. In the twin piers test, the shift occurred before the vanes’ location. In the single pier test, this shift in direction happened between the pier and the vanes. In both tests, the maximum velocity path was oriented towards the outer bank from the downstream half of the bend; nevertheless, closer to the bend’s end, its orientation was further strengthened towards the outer bank wall.
The maximum velocity path at almost mid-depth is analysed in Fig. 18b. Considering this figure, with installation of the single pier and the vanes, the maximum velocity path went towards mid-channel from the bend entrance, and oriented towards the outer bank upon reaching the vanes’ location. Its geometric location was completely in the vicinity of the outer bank wall in the downstream half of the bend because the longitudinal pressure gradient near the outer bank became negative. As shown in this figure, in the twin piers test, an abrupt shift in the maximum velocity path occurred upon reaching the upstream side of the vanes so that the maximum resultant velocity path shifted from the inner bank towards the vicinity of Vo (the vane closer to the outer bank), and afterwards, it oriented towards the outer bank.
Figure 18c depicts the maximum resultant velocity path at the water surface level. In P-2V25, before reaching the vanes, from approximately 60 degrees, the velocity path abruptly shifted directions towards the outer bank to the end of the bend. The same results were also observed in the study carried out by Abdi Chooplou et al. (2018), where the maximum velocity deviated from the single pier after distancing away from the initial bed level and was transported to the upstream side of the submerged vanes. In 2PT-2V25, the maximum velocity path from the bend entrance mildly advanced towards mid-channel, and had an abrupt shift at the site of the vanes. Then its location was oriented towards the Po pier (the pier at the outer bank side). Thereafter, it was directed towards the outer bank.
As a general conclusion to be made, the geometric location of the maximum resultant velocity occurred in the upstream half of the bend near the inner bank, it shifted orientation at the bend’s centre, and it was oriented towards the outer bank in the downstream half. There is a similarity between this study’s results on the maximum resultant velocity’s geometric location in the single pier test and the results of the single pier test of Abdi Chooplou et al. (2018), where the difference was only found in the vanes’ submergence as well as their location. However, the comparison of the geometric location of the maximum resultant velocity in the single pier and the twin piers tests in this study showed difference only in the bend’s centre (considering every parameter related to the submerged vanes). Hence, it can be concluded that such a difference stems from installation of the group of piers.
Conclusions
This study explored the flow pattern around a single pier and a group of twin piers transverse to the flow direction together with installation of submerged vanes at the upstream side in a 180° sharp bend. The velocity components around the piers were compared with each other, and the most important findings of this comparison are as follows.
In both experiments at plan section at the level near the bed, the highest tangential velocity in the first half of the bend occurred near the inner bank and in the second half of the bend, it occurred near the outer bank. In the centre of channel, the flow was influenced by the piers, causing a decrease in tangential velocities. The maximum tangential velocities in both experiments were approximately equal. However, at the water surface level, the difference in tangential velocities increased around the vanes up to the location of the piers.
In the twin piers test, the radial velocity values at plan section at the level near the bed increased compared to that in the single pier test and decreased by distancing away from the initial bed level; however, the tangential and vertical velocity values in both tests showed very little difference.
By comparing the transverse velocity distributions, radial velocities in the second half of the bend in experiment 2PT-2V25 increased compared to experiment P-2V25, which was accompanied by a higher turbulence intensity.
The highest negative radial velocities were obtained near the inner bank in 2PT-2V25 experiment. The radial velocity towards the inner bank was predominant in the mid-channel up to approximately 30% of the flow depth from the initial bed, causing the interaction of this flow with the longitudinal flow, resulting in lateral flows and consequently scouring.
The changes in velocities became steadier when approaching the water surface. Consequently, the flow near the bed was accompanied by more turbulence and agitation, with maximum turbulence intensity occurring at the location of the piers and vanes.
In the single pier and twin piers transverse to the flow tests involving two vanes with 25% submergence, the maximum vorticity was obtained at the central position of the channel in the vicinity of the piers. The maximum secondary flow power in these tests occurred within the approximate range of 80.5 to 91 degrees in the bend corresponding to 56 and 63D from the bend entrance.
In both tests, the maximum Reynolds stress value of \(\tau_{yx}\) was found around the vanes, and its minimum occurred behind the bridge piers. The maximum Reynolds stress of \(\tau_{zy}\) was observed behind the bridge pier in the single pier test and around the pier close to the inner bank in the twin piers test. In both tests, the maximum \(\tau_{zx}\) value within the range from the pier to the vanes took place near the vane closer to the inner bank.
In both tests, the geometric location of the maximum resultant velocity in the bend’s upstream half was near the inner bank owing to the longitudinal pressure gradient. At the bend’s centre, upon approaching the piers and submerged vanes, it shifted orientation in the downstream half towards the outer bank. In the single pier test, away from the initial bed level, the geometric location of the maximum velocity was transported to the upstream side of the vanes.
In general, installation of the piers and vanes at the bend’s centre caused fluctuations in the distribution of longitudinal, lateral, and vertical velocity components so that the flow pattern in the upstream half of the bend up to the area before the submerged vanes was the same in both tests. However, at the bend’s centre and its downstream side, the flow pattern was affected by the presence of the piers and vanes. The disorderly pattern around the piers and the vanes, and also the occurrence of the maximum secondary flow pattern at this point, represent the general findings of this study.
References
Abdi Chooplou C, Vaghefi M, Meraji SH (2018) Study of streamlines under the influence of displacement of submerged vanes in channel width, and at the upstream area of a cylindrical bridge pier in a 180 degree sharp bend. J Hydraul Struct 4:55–74. https://doi.org/10.22055/JHS.2018.25552.1071
Abdulkathum S, Al-Shaikhli HI, Al-Abody AA, Hashim TM (2023) Statistical analysis approaches in scour depth of bridge piers. Civ EngJ 9:143–153. https://doi.org/10.28991/CEJ-2023-09-01-011
Akbari M, Vaghefi M (2017) Experimental investigation on streamlines in a 180º sharp bend. Acta Sci Technol 39:425–432. https://doi.org/10.4025/actascitechnol.v39i4.29032
Akbari M, Vaghefi M, Chiew YM (2021) Effect of T-shaped spur dike length on mean flow characteristics along a 180° sharp bend. J Hydrol Hydromech 69:98–107. https://doi.org/10.2478/johh-2020-0045
Akhtari AA, Seyedashraf O (2018) Experimental and numerical investigation on vanes’ effects on the flow characteristics in sharp 60° bends. KSCE J Civ Eng 22:1484–1495. https://doi.org/10.1007/s12205-017-1743-y
Aminoroayaie Yamini O, Mousavi SH, Kavianpour MR, Movahedi A (2018) Numerical modeling of sediment scouring phenomenon around the offshore wind turbine pile in marine environment. Environ Earth Sci 77:1–15. https://doi.org/10.1007/s12665-018-7967-4
Asadollahi M, Vaghefi M, Tabibnejad Motlagh MJ (2021) Experimental and numerical comparison of flow and scour patterns around a single and triple bridge piers located at a 180° sharp bend. Sci Iran 28:1–14. https://doi.org/10.24200/sci.2019.5637.1391
Azizi S, Farsadizadeh D, Arvanaghi H, Abbaspour A (2016) Numerical simulation of flow pattern around the bridge pier with submerged vanes. J Hydraul Struct 2:46–61. https://doi.org/10.22055/jhs.2016.12856
Blanckaert K, Graf WH (2001) Mean flow and turbulence in open-channel bend. J Hydraul Eng 127:835–847. https://doi.org/10.1061/(ASCE)0733-9429(2001)127:10(835)
Chauhan V, Chavan R, Singhal G D (2022) Numerical modeling for optimization of the aspect ratio of submerged vanes for the purpose of sediment deflection in rivers. 9th ISHS. https://doi.org/10.26077/b079-8746
Chiew YM, Melville BW (1987) Local scour around bridge piers. J Hydraul Res 25:15–26. https://doi.org/10.1080/00221688709499285
Chooplou A, Vaghefi M (2019) Experimental study of the effect of displacement of vanes submerged at channel width on distribution of velocity and shear stress in a 180 degree bend. J Appl Fluid Mech 12:1417–1428. https://doi.org/10.29252/jafm.12.05.29329
Das S, Das R, Mazumdar A (2013a) Circulation characteristics of horseshoe vortex in scour region around circular piers. Water Sci Eng 6:59–77. https://doi.org/10.3882/j.issn.1674-2370.2013.01.005
Das S, Das R, Mazumdar A (2013b) Comparison of characteristics of horseshoe vortex at circular and square piers. Res J Appl Sci 5:4373–4387. https://doi.org/10.19026/rjaset.5.4432
Graf WH, Istiarto I (2002) Flow pattern in the scour hole around a cylinder. J Hydraul Res 40:13–20. https://doi.org/10.1080/00221680209499869
Guan D, Chiew YM, Wei M, Hsieh SC (2019) Characterization of horseshoe vortex in a developing scour hole at a cylindrical bridge pier. Int J Sediment Res 34:118–124. https://doi.org/10.1016/j.ijsrc.2018.07.001
Han SS, Ramamurthy AS, Biron PM (2011) Characteristics of flow around open channel 90 bends with vanes. J Irrig Drain Eng 137:668–676. https://doi.org/10.1061/(ASCE)IR.1943-4774.0000337
Hassan WH, Shabat NA (2023) Numerical investigation of the optimum angle for open channel junction. Civ Eng J. https://doi.org/10.28991/CEJ-2023-09-05-07
Marelius F (2001) Experimental investigation of vanes as a means of beach protection. Coast Eng 42:1–16. https://doi.org/10.1016/S0378-3839(00)00044-2
Moghanloo M, Vaghefi M, Ghodsian M (2020) Experimental investigation on the effect of increasing the collar thickness on the flow pattern around the oblong pier in 180 sharp bend with balanced bed. J Appl Fluid Mech 13:245–260. https://doi.org/10.29252/jafm.13.01.30164
Odgaard AJ (2009) River training and sediment management with submerged vanes. ASCE. https://doi.org/10.1061/9780784409817
Odgaard AJ, Wang Y (1991) Sediment management with submerged vanes. II: applications. J Hydraul Eng 117:284–302. https://doi.org/10.1061/(ASCE)0733-9429(1991)117:3(284)
Odgaard AJ (2017) River management with submerged vanes. River Syst Anal Manag. https://doi.org/10.1007/978-981-10-1472-7_13
Raudkivi AJ, Ettema R (1983) Clear-water scour at cylindrical piers. J Hydraul Eng 109:338–350. https://doi.org/10.1061/(ASCE)0733-9429(1983)109:3(33
Safaripour N, Vaghefi M, Mahmoudi A (2022) Experimental study of the effect of submergence ratio of double submerged vanes on topography alterations and temporal evaluation of the maximum scour in a 180° bend with a bridge pier group. Int J River Basin Manag 20:427–441. https://doi.org/10.1080/15715124.2020.1837144
Sharma H, Ahmad Z (2020) Turbulence characteristics of flow past submerged vanes. Int J Sediment Res 35:42–56. https://doi.org/10.1016/j.ijsrc.2019.07.002
Shukry A (1950) Flow around bends in an open flume. Trans Am Soc Civil Eng 115:751–778. https://doi.org/10.1061/TACEAT.0006426
Sinha SK, Marelius F (2000) Analysis of flow past submerged vanes. J Hydraul Res 38:65–71. https://doi.org/10.1080/00221680009498360
Tan SK, Yu G, Lim SY, Ong MC (2005) Flow structure and sediment motion around submerged vanes in open channel. J Waterw Port Coast Ocean Eng 131:132–136. https://doi.org/10.1061/(ASCE)0733-950X(2005)131:3(132)
Taşar B, Üneş F, Gemici E (2023) Laboratory and numerical investigation of the 2-array submerged vanes in meandering open channel. Math Biosci Eng 20:3261–3281. https://doi.org/10.3934/mbe.2023153
Vaghefi M, Akbari M, Fiouz AR (2016) An experimental study of mean and turbulent flow in a 180 degree sharp open channel bend: secondary flow and bed shear stress. KSCE J Civ Eng 20:1582–1593. https://doi.org/10.1007/s12205-015-1560-0
Vaghefi M, Ghodsian M, Akbari M (2017) Experimental investigation on 3D flow around a single T-shaped spur dike in a bend. Period Polytech Chem Eng 61:462–470. https://doi.org/10.3311/PPci.7999
Vaghefi M, Moradi S, Abdi Chooplou C (2021) Numerical study of bed topography variations owing to placement of triad series of vertical bridge piers installed in a 180° bend with different relative radii using SSIIM numerical model. Iran J Sci Technol Trans 45:1971–1988. https://doi.org/10.1007/s40996-020-00559-7
Vaghefi M, Zarei E, Ahmadi G, Behroozi AM (2023) Experimental analysis of submerged vanes’ configuration for mitigating local scour at piers in a sharp bend: influence of quantity, length, and orientation. Ocean Eng 289:116267. https://doi.org/10.1016/j.oceaneng.2023.116267
White FM (1986) Fluid mechanics. McGraw-Hill International Book Co, USA
Funding
The authors received no funding for this work.
Author information
Authors and Affiliations
Contributions
The authors declare that they have contribution in the preparation of this manuscript.
Corresponding author
Ethics declarations
Conflict of interest
The authors have no relevant financial or non-financial interests to disclose.
Ethical approval
This work has not been published elsewhere nor is it currently under consideration for publication elsewhere.
Consent to participate
The authors have read the final manuscript, have approved the submission to the journal and have accepted full responsibilities pertaining to the manuscript’s delivery and contents.
Consent to publish
The authors agree to publish this manuscript upon acceptance.
Additional information
Publisher's Note
Springer Nature remains neutral with regard to jurisdictional claims in published maps and institutional affiliations.
Rights and permissions
Open Access This article is licensed under a Creative Commons Attribution 4.0 International License, which permits use, sharing, adaptation, distribution and reproduction in any medium or format, as long as you give appropriate credit to the original author(s) and the source, provide a link to the Creative Commons licence, and indicate if changes were made. The images or other third party material in this article are included in the article's Creative Commons licence, unless indicated otherwise in a credit line to the material. If material is not included in the article's Creative Commons licence and your intended use is not permitted by statutory regulation or exceeds the permitted use, you will need to obtain permission directly from the copyright holder. To view a copy of this licence, visit http://creativecommons.org/licenses/by/4.0/.
About this article
Cite this article
Safaripour, N., Vaghefi, M. & Mahmoudi, A. An experimental comparison of 3D velocity components around single and twin piers installed in a sharp bend under the influence of upstream implemented vanes. Appl Water Sci 14, 107 (2024). https://doi.org/10.1007/s13201-024-02177-4
Received:
Accepted:
Published:
DOI: https://doi.org/10.1007/s13201-024-02177-4