Discovery of new eremophilanes from the marine-derived fungus Emericellopsis maritima BC17 by culture conditions changes: evaluation of cytotoxic and antimicrobial activities
- 1Departamento de Química Orgánica, Facultad de Ciencias, Universidad de Cádiz, Cádiz, Spain
- 2Instituto de Investigación en Biomoléculas (INBIO), Universidad de Cádiz, Cádiz, Spain
- 3Departamento de Química Física, Facultad de Ciencias, Universidad de Cádiz, Cádiz, Spain
- 4Centro de Excelencia en Investigación de Medicamentos Innovadores en Andalucía, Fundación MEDINA, Granada, Spain
In our previous studies, the marine-derived fungus Emericellopsis maritima BC17 was found to produce new eremophilane-type sesquiterpenoids on solid media. In order to explore its potential to produce more metabolites, E. maritima BC17 was subjected to a one strain-many compounds (OSMAC) analysis leading to the discovery of three new eremophilanes (1-3) and fourteen known derivatives (4-17) in the liquid media Czapek Dox and PDB. Their structures were established by extensive analyses of the 1D and 2D NMR, and HRESIMS data, as well as ECD data for the assignment of their absolute configurations. Antitumoral and antimicrobial activities of the isolated metabolites 1, 3, 11, and 15 were investigated. PR toxin 3-deacetyl (15) exhibited cytotoxic activity against HepG2, MCF-7, A549, A2058 and Mia PaCa-2 human cancer cell lines with IC50 values ranging from 2.5 to 14.7 µM. In addition, 15 exhibited selective activity against methicillin-sensitive Staphylococcus aureus ATCC29213 at the highest concentration tested of 128 µg/mL.
1 Introduction
Marine fungi strains can be found across diverse habitats holding a notable ecological importance due to their ability to produce a wide range of metabolites (Grossart et al., 2019; Hafez Ghoran et al., 2022), including peptides, terpenes, lactones, steroids, alkaloids and polyketides (Jin et al., 2016).
In recent decades, the interest in marine fungi as chemical producers of bioactive molecules has increased significantly due to the isolation of new compounds with potential pharmaceutical applications (Xu et al., 2015; Jin et al., 2016; Pang et al., 2016; Khalifa et al., 2019; Wang et al., 2022), playing a crucial role in discovering and developing new drugs (Agrawal et al., 2023).
Given the increasing need to find and develop new bioactive molecules to combat global health problems such as microbial resistance and the rising incidence of cancer (Christaki et al., 2020; Sung et al., 2021), marine-derived fungi have proven to be a promising source of novel bioactive metabolites. Additionally, cytotoxic and antibacterial activities are the most commonly reported bioactivities of natural products from these fungi (Jin et al., 2016). In fact, halimide, a potent cytotoxic diketopiperazine isolated from the marine-derived fungus Aspergillus ustus, has led to the synthesis of plinabulin (NPI-2358), which is currently undergoing phase II/III clinical trials for cancer treatment (Blayney et al., 2020, 2022).
Traditional methodologies for the chemical exploration of new bioactive natural products are currently considered inefficient due to recurrent rediscovery of known compounds or the limited yields obtained under laboratory conditions. In this sense, one of the most widely used strategies to exploit the chemical diversity produced by microorganisms involves the manipulation of culture conditions, such as variation of medium composition, temperature, and pH. This approach is known as the “one strain many compounds” (OSMAC) approach (Bode et al., 2002). OSMAC strategy, which attempts to activate silenced biosynthetic pathways, has already been successfully employed in marine-derived fungal strains leading to the isolation of a wide range of compounds with interesting antimicrobial and cytotoxic activities (Pinedo-Rivilla et al., 2022).
Emericellopsis, a genus of Ascomycota fungi within the order Hypocreales, has a wide environmental amplitude and universal distribution (Grum-Grzhimaylo et al., 2013). According to the different phylogeny and ecology, it has been classified into two distinct groups, specifically marine and terrestrial clades (Zuccaro et al., 2004).
Emericellopsis spp. are ubiquitous in marine environments and can survive extreme conditions, including temperature, pressure, salinity, and pH (Grum-Grzhimaylo et al., 2013). These fungi have also been isolated from different marine macroalgae and sponges (Wiese et al., 2011; Gonçalves et al., 2020) and have previously been studied for their ability to produce peptides with antifungal (Kuvarina et al., 2021), antitumoral (Rogozhin and Sadykova, 2019), and antibiotic activities (Inostroza et al., 2018; Agrawal and Saha, 2022).
Specifically, E. maritima has previously been isolated from Atlantic sponges and the deep-sea (Batista-García et al., 2017; Bovio et al., 2018). However, no secondary metabolites from this species had been published, making its study an important starting point as a potential source of bioactive compounds.
The strain E. maritima BC17 was isolated from sediments collected along an intertidal gradient of the inner Bay of Cádiz, Spain (Virués-Segovia et al., 2023). Four new eremophilane-type sesquiterpenes, together with thirteen known derivatives were found in the culture broth of this fungus on different solid media (Virués-Segovia et al., 2023).
Eremophilanes have been reported to exhibit a wide variety of bioactivities such as phytotoxins, antimicrobials, protein inhibitors, immunomodulators and cytotoxins. Indeed, these sesquiterpenes have previously been isolated from marine fungi such as Penicillium copticola (Zhang et al., 2022), Xylaria sp. BL321 (Song et al., 2012), Penicillium sp. PR19N-1 (Wu et al., 2013; Lin et al., 2014) or Aspergillus sp. SCSIOW2 (Wang et al., 2016), among others, showing an interesting diversity of bioactive compounds (Ebel, 2010).
With the aim of continuing the study of its chemical potential, in this work E. maritima BC17 was further investigated using liquid culture media following the OSMAC strategy. Consequently, three previously undescribed eremophilanes (1-3) and fourteen known derivatives (4-17) were isolated using the liquid media Czapek Dox and potato dextrose broth (PDB), both under surface culture and shaking conditions. Additionally, the antimicrobial and cytotoxic activities of compounds 1, 3, 11, and 15 are reported.
2 Materials and methods
2.1 General experiment procedures
Melting points were measured with a Reichert-Jung Kofler block. ECD spectra were recorded on a JASCO J-1500 CD spectrometer. Optical rotations were determined with a JASCO P-2000 polarimeter. IR spectra were recorded on a PerkinElmer Spectrum BX FT-IR spectrophotometer and reported as wavenumber (cm—1). TLC was performed on Merck Kiesegel 60 Å F254, 0.25 mm thick. Silica gel 60 PF254 (60-100 mesh, VWR) was used for column chromatography. HPLC was performed with a Hitachi/Merck L-6270 apparatus equipped with an UV-VIS detector (L 4250) and a differential refractometer detector (RI-7490). LiChroCART LiChrospher Si 60 (5 µm, 250 mm × 4 mm), LiChroCART LiChrospher Si 60 (10 µm, 250 mm × 10 mm), and ACE 5 SIL (5 µm, 250 mm × 4.6 mm id) columns were used for isolation experiments. 1H and 13C NMR measurements were recorded on Bruker 400, 500, and 700 MHz spectrometers with SiMe4 as the internal reference. Chemical shifts are expressed in ppm (δ), referenced to CDCl3 (Eurisotop, Saint-Aubiu, France, δH 7.25, δC 77.0) and CD3OD (Eurisotop, Saint-Aubiu, France, δH 3.30, δC 49.0). COSY, HSQC, HMBC, and NOESY experiments were performed using standard Bruker pulse sequence. NMR assignments were made using a combination of 1D and 2D techniques. High-Resolution Mass Spectroscopy (HRMS) was performed either with a double-focusing magnetic sector mass spectrometer in a QTOF mass spectrometer in the positive-ion ESI mode.
2.2 Fungal material
The strain used in this work, E. maritima BC17, was isolated from sediment samples collected along an intertidal gradient of Bay of Cádiz, Spain (Virués-Segovia et al., 2023). This culture was deposited at the University of Cádiz, Mycological Herbarium Collection (UCA). Conidial stock suspensions were maintained viable in 80% glycerol at -40°C.
2.3 Culture and extraction conditions
E. maritima BC17 strain was grown in Petri dishes with potato dextrose agar (PDA, Condalab, Madrid, Spain) medium for one week at 25°C under white light (day light lamp).
For surface cultures, 26 Roux bottles containing 150 mL of PDB (Condalab, Madrid, Spain) or Czapek-Dox medium (50 g glucose, 1 g yeast extract, 5 g K2HPO4, 2 g NaNO3, 0.5 g MgSO4·7H2O, 0.01 g FeSO4·7H2O, 1L of water and pH adjusted to 6.5-7.0) were inoculated with 5 mycelium plugs (9 mm) from a 7 days-old culture. The bottles were incubated at 25°C under white light for 14 and 28 days.
For shaken cultures, the strain E. maritima BC17 was fermented in 1.4 L of Czapek-Dox or 3.2 L of PDB liquid media (200 mL per flask) for 14 days at 25°C and 120 rpm under continuous white light. Each Erlenmeyer flask was inoculated with 5 PDA discs (9 mm) from a 7 days-old culture. Fermentation continued at 25°C under continuous white light for 14 or 28 days.
Once the fermentation was finished, the mycelium was filtered. The broth was extracted three times with ethyl acetate and the organic extract dried over dry Na2SO4. The solvent was then evaporated and the residue chromatographed, first on a silica gel column and then by HPLC with an increasing gradient of ethyl acetate to n-hexane.
2.4 Isolation and characterization of metabolites
2.4.1 Surface culture fermentation
Chromatography of the extract fermented in Czapek Dox medium for 14 days (746 mg) gave, in addition to the known compounds 2-phenylethanol (22.0 mg), tyrosol (10.0 mg), 4 (3.5 mg), 5 (3.8 mg), 6 (2.0 mg), 7 (1.0 mg), and 8 (49.1 mg), the new metabolites 1 (1.7 mg), 2 (0.8 mg), and 3 (1.0 mg). Purification of the extract fermented for 28 days (316 mg) afforded the known compounds 2-phenylethanol (31.0 mg), tyrosol (32.0 mg), 4 (10.9 mg), 5 (10.6 mg), 6 (2.9 mg), 8 (1.8 mg), 9 (1.0 mg), and 10 (1.5 mg), and the new metabolites 2 (1.2 mg), and 3 (2.0 mg) (Figure 1).
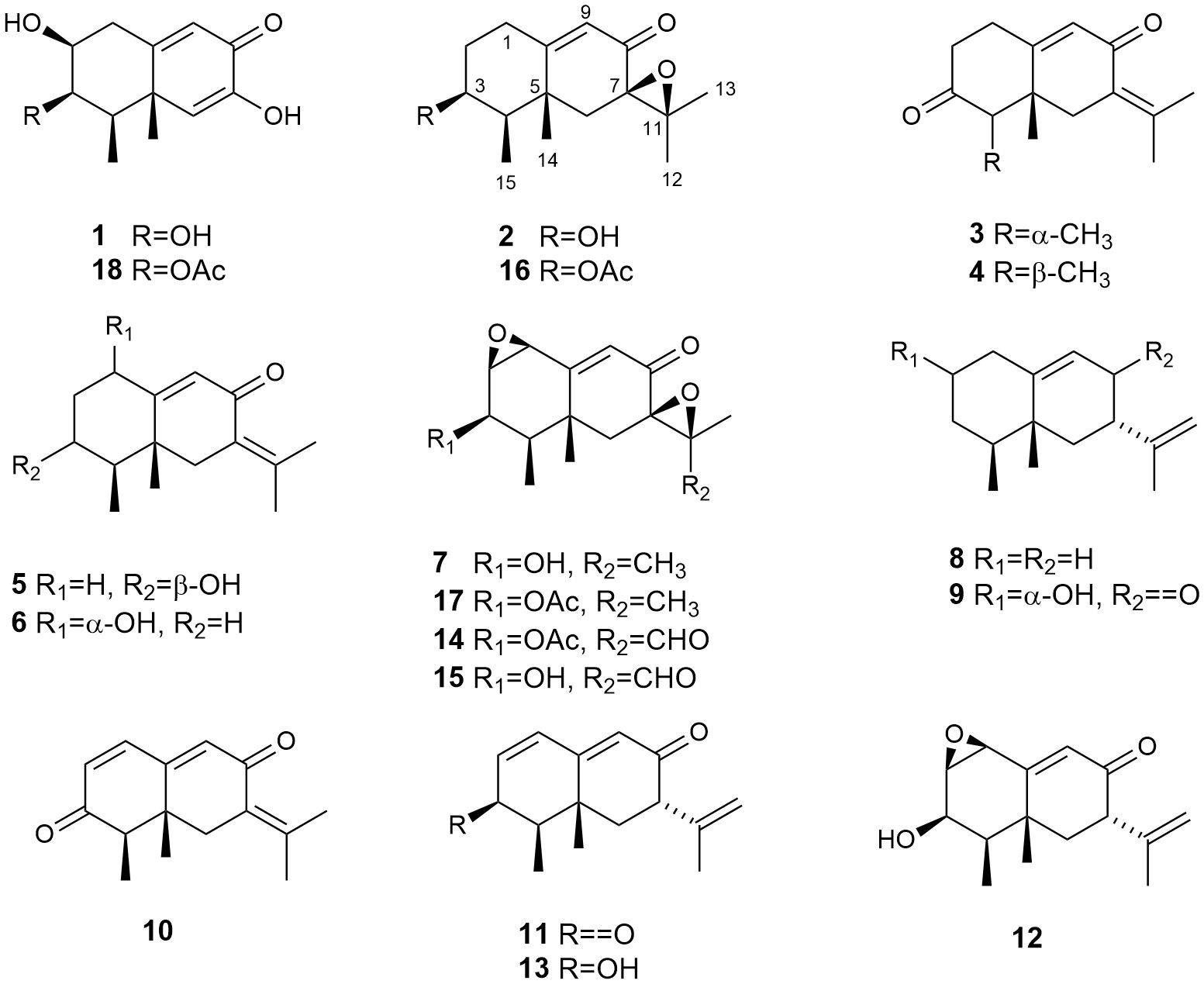
Figure 1 Eremophilanes (1-17) isolated from the strain Emericellopsis maritima BC17 cultivated in liquid culture media.
3-Deacetyl guignarderemophilane A (1): purified through semipreparative HPLC (n-hexane: EtOAc : Acetone 55:45:5, flow 2.0 mL/min, tR = 31 min). White solid; mp 64.7°C; -33.7° (c 0.17, MeOH); ECD (MeOH) λ (Δϵ) 214 (5.74), 256 (-2.71), 288 (-2.03) nm; IR νmax (cm-1) 3447, 2932, 1637, 1448, 655; HRMS(ESI+) calcd. for C12H16O4Na [M+Na]+ 247.0946, found 247.0933; 1H and 13C NMR data in Table 1; gCOSY (selected correlations) H-1α → H-1β, H-2α; H-1β → H-1α, H-2α, H-9; H-2α → H-1β, H-1α, H-3α; H-3α → H-2α, H-4α; H-4α → H-3α, Me-15β; H-9 → H-1β; Me-15β → H-4α (Supplementary Figure S3); gHMBC (selected correlations) H-1α → C-3, C-5, C-9, C-10; H-1β → C-2, C-9, C-10; H-4α → C-5, C-14, C-15; H-6 → C-4, C-7, C-8, C-10, C-14; H-9 → C-1, C-5, C-7; Me-14β → C-4, C-5, C-6, C-10; Me-15β → C-3, C-4, C-5 (Supplementary Figure S5).
(3S,4R,5R,7R)-3-Hydroxy-7(11)-epoxyeremophil-9-en-8-one (2): purified through semipreparative HPLC (n-hexane: EtOAc : Acetone 65:30:5, flow 2.5 mL/min, tR = 51 min). Colourless oil. -25.4° (c 1.3, CHCl3); ECD (MeOH) λ (Δϵ) 205 (0.76), 220 (-3.12), 268 (-2.56), 331 (2.31) nm; IR νmax (cm-1) 3496, 2850, 1674; HRMS(ESI+) calcd. for C15H22O3Na [M+Na]+ 273.1467, found 273.1446; 1H and 13C NMR data in Table 1; gCOSY (selected correlations) H-1β → H-1α, H-2α, H-2β, H-9; H-2α → H-1β, H-1α, H-2β, H-3α; H-3α → H-2α, H-4α; H-4α → Me-15β; H-9 → H-1β; Me-15β → H-4α (Supplementary Figure S10); gHMBC (selected correlations) H-1β → C-9, C-10; H2-6 → C-4, C-5, C-7, C-10, C-11, C-14; H-9 → C-1, C-5, C-7; Me-12 → C-7, C-11, C-13; Me-13 → C-7, C-11, C-12; Me-14β → C-4, C-5, C-6, C-10; Me-15β → C-3, C-4, C-5 (Supplementary Figure S12).
4-Epiisopetasone (3): purified through semipreparative HPLC (n-hexane:EtOAc 80:20, flow 2.5 mL/min, tR = 83 min). Colourless oil. +83.3° (c 0.3, CHCl3); ECD (MeOH) λ (Δϵ) 213 (2.74), 246 (6.13), 282 (-2.80) nm; IR νmax (cm-1) 2924, 1717, 1660, 1458; HRMS(ESI+) calcd. for C15H21O2 [M+H]+ 233.1542, found 233.1543; 1H and 13C NMR data in Table 1; gCOSY (selected correlations) H-1β → H-1α, H-2, H-9; H-2 → H-1β, H-1α; H-4β → Me-15α; H-9 → H-1β; Me-15α → H-4β (Supplementary Figure S17); gHMBC (selected correlations) H-1β → C-2, C-9, C-10; H-4β → C-5, C-14, C-15; H-9 → C-1, C-5, C-7; Me-12 → C-7, C-11, C-13; Me-13 → C-7, C-11, C-12; Me-14β → C-4, C-5, C-6, C-10; Me-15α → C-3, C-4, C-5 (Supplementary Figure S19).
Isopetasone (4): purified through semipreparative HPLC (n-hexane:EtOAc 85:15, flow 3.0 mL/min, tR = 62 min). +31° (c 0.06, CHCl3). 1H NMR (500 MHz, CDCl3) δ 5.92 (dd, 1H, J = 1.8, 0.9 Hz, H-9), 2.82 (d, 1H, J = 13.9, H-6β), 2.79 – 2.68 (m, 2H, H-1), 2.60 – 2.48 (m, 2H, H-2), 2.57 (q, 1H, J = 6.6 Hz, H-4), 2.45 (br d, 1H, J = 13.9 Hz, H-6α), 2.11 (d, 3H, J = 1.9 Hz, H-12)a, 1.86 (d, 3H, J = 1.2 Hz, H-13)a, 1.08 (d, 3H, J = 6.7 Hz, H-15), 0.95 (s, 3H, H-14). 13C NMR (125 MHz, CDCl3) δ 210.2 (C-3, C), 191.0 (C-8, C), 162.8 (C-10, C), 144.6 (C-11, C), 127.7 (C-9, CH), 126.7 (C-7, C), 52.6 (C-4, CH), 44.6 (C-5, C), 40.7 (C-6, CH2), 39.8 (C-2, CH2), 31.1 (C-1, CH2), 22.7 (C-13, CH3)b, 22.3 (C-12, CH3)b, 18.5 (C-14, CH3), 7.5 (C-15, CH3). a,b Interchangeable assignments.
(+)-3-Epiisopetasol (5): purified through semipreparative HPLC (n-hexane: EtOAc : Acetone 80:20, flow 2.5 mL/min, tR = 86 min). +110° (c 0.11, CHCl3). 1H NMR (500 MHz, CDCl3) δ 5.77 (d, J = 1.9 Hz, 1H, H-9), 3.93 (q, J = 2.8 Hz, 1H, H-3α), 2.87 (d, J = 13.0 Hz, 1H, H-6β), 2.76 (tdd, J = 14.4, 5.1, 1.9 Hz, 1H, H-1β), 2.12 (ddd, J = 14.4, 4.4, 2.6 Hz, 1H, H-1α), 2.09 (s, 3H, H-12)c, 2.08 (br d, 1H, J = 13.0 Hz, H-6α), 2.00 (ddt, J = 14.0, 5.1, 2.6 Hz, 1H, H-2β), 1.83 (d, J = 1.0 Hz, 3H, H-13)c, 1.69 (tdd, J = 14.0, 4.4, 2.8 Hz 1H, H-2α), 1.55 (qd, J = 7.3, 2.8 Hz, 1H, H-4), 1.15 (s, 3H, H-14),1.12 (d, J = 7.3 Hz, 3H, H-15). 13C NMR (125 MHz, CDCl3) δ 192.2 (C-8, C), 168.3 (C-10, C), 143.1 (C-11, C), 127.9 (C-7, C), 126.1 (C-9, CH), 71.3 (C-3, CH), 45.4 (C-4, CH), 41.9 (C-6, CH2), 41.4 (C-5, C), 33.7 (C-2, CH2), 26.7 (C-1, CH2), 22.6 (C-12, CH3)d, 22.1 (C-13, CH3)d, 18.9 (C-14, CH3), 12.1 (C-15, CH3). c,d Interchangeable assignments.
1α-Hydroxydehydrofukinone (6): purified through semipreparative HPLC (n-hexane:EtOAc 72:28, flow 3.0 mL/min, tR = 39 min). +47° (c 0.05, CHCl3). 1H NMR (500 MHz, CDCl3) δ 5.84 (s, 1H, H-9), 4.33 (t, J = 3.0 Hz, 1H, H-1β), 2.90 (d, J = 14.2 Hz, 1H, H-6β), 2.12 (br d, J = 14.2 Hz, H-6α), 2.11 (s, 3H, H-12)e, 1.99 (dq, J = 13.9, 3.0 Hz, 1H, H-2α), 1.85 (s, 3H, H-13)e, 1.83 (qd, J = 13.4, 3.3 Hz, 1H, H-3α), 1.67 (tt, J = 13.9, 3.5 Hz, 1H, H-2β), 1.48 (dqd, J = 13.4, 6.9, 3.3 Hz, 1H, H-4), 1.39 (dq, J = 13.4, 3.5 Hz, 1H, H-3β), 1.14 (s, 3H, H-14), 0.98 (d, J = 6.8 Hz, 3H, H-15). 13C NMR (125 MHz, CDCl3) δ 192.4 (C-8, C), 165.6 (C-10, C), 144.3 (C-11, C), 129.1 (C-9, CH), 128.1 (C-7, C), 72.7 (C-1, CH), 42.3 (C-6, CH2), 42.3 (C-4, CH), 41.0 (C-5, C), 32.8 (C-2, CH2), 24.8 (C-3, CH2), 22.8 (C-12, CH3)f, 22.4 (C-13, CH3)f, 18.1 (C-14, CH3), 15.5 (C-15, CH3). e,f Interchangeable assignments.
Eremofortin A alcohol (7): purified through semipreparative HPLC (n-hexane:EtOAc 60:40, flow 3.0 mL/min, tR = 31 min). +71° (c 0.06, CHCl3). 1H NMR (600 MHz, CDCl3) δ 6.39 (s, 1H, H-9), 4.11 (dt, J = 9.9, 5.1 Hz, 1H, H-3), 3.87 (dd, J = 5.1, 3.6 Hz, 1H, H-2), 3.76 (d, J = 3.6 Hz, 1H, H-1), 2.09 (dd, J = 14.7, 1.0 Hz, 1H, H-6α), 1.92 (d, J = 14.7 Hz, 1H, H-6β), 1.54 (m, 1H, H-4), 1.52 (s, 3H, H-13), 1.38 (s, 3H, H-12), 1.28 (s, 3H, H-14), 1.11 (d, J = 7.1 Hz, 3H, H-15). 13C NMR (150 MHz, CDCl3) δ 194.0 (C-8, C), 160.5 (C-10, C), 131.9 (C-9, CH), 67.7 (C-3, CH), 64.6 (C-11, C), 62.3 (C-7, C), 58.0 (C-1, CH), 58.0 (C-2, CH), 44.0 (C-4, CH), 42.1 (C-6, CH2), 37.1 (C-5, C), 23.4 (C-14, CH3), 21.5 (C-12, CH3), 19.4 (C-13, CH3), 10.6 (C-15, CH3).
(+)-Aristolochene (8): purified through silica gel column (n-hexane:EtOAc 100:0). +47° (c 0.23, CHCl3). 1H NMR (500 MHz, CDCl3) δ 5.31 (dt, J = 5.6, 2.0 Hz, 1H, H-9), 4.71 (m, 2H, H-13), 2.21 (m, 1H, H-7β), 2.13 (m, 1H, H-2α)g, 2.05 – 1.97 (m, 2H, H-2β, H-8α)g,i, 1.87 (dddd, J = 16.9, 11.6, 3.4, 2.0 Hz, 1H, H-8β)i, 1.77 (dt, J = 12.7, 2.3 Hz, 1H, H-6α)h, 1.74 (t, J = 1.1 Hz, 3H, H-12), 1.73 (m, 1H, H-3β)j, 1.42 (m, 1H, H-1α)k, 1.35 (td, J = 12.2, 11.7, 2.8 Hz, 1H, H-1β)k, 1.31 – 1.22 (m, 2H, H-3α, H-4)j, 1.16 (td, J = 12.7, 0.9 Hz, 1H, H-6β)h, 0.97 (s, 3H, H-14), 0.84 (d, J = 6.6 Hz, 3H, H-15). 13C NMR (125 MHz, CDCl3) δ 150.6 (C-11, C), 144.4 (C-10, C), 118.8 (C-9, CH), 108.3 (C-13, CH2), 44.2 (C-4, CH), 43.3 (C-6, CH2), 38.7 (C-5, C), 37.8 (C-7, CH), 32.6 (C-2, CH2), 31.3 (C-8, CH2), 31.1 (C-1, CH2), 27.8 (C-3, CH2), 20.8 (C-12, CH3), 18.1 (C-14, CH3), 15.7 (C-15, CH3). g–k Interchangeable assignments.
7-Hydroxy-4a,5-dimethyl-3-prop-1-en-2-yl-3,4,5,6,7,8-hexahydronaphthalen-2-one (9): purified through analytical HPLC (n-hexane:EtOAc 60:40, flow 1.0 mL/min, tR = 32 min). +90° (c 0.12, CHCl3). 1H NMR (500 MHz, CDCl3) δ 5.81 (d, J = 2.0 Hz, 1H, H-9), 4.97 (p, J = 1.5 Hz, 1H, H-13), 4.81 (dq, J = 1.8, 0.8 Hz, 1H, H-13’), 4.20 (br s, 1H, H-2β), 3.13 (dd, J = 13.8, 5.1 Hz, 1H, H-7), 2.59 (ddd, J = 15.2, 3.4, 2.1 Hz, 1H, H-1β), 2.37 (m, 1H, H-1α), 2.05 – 1.89 (m, 3H, H-4, H-6), 1.73 (dd, J = 1.5, 0.8 Hz, 3H, H-12), 1.69 (ddd, J = 7.9, 3.0, 1.7, 2H, H-3), 1.16 (s, 3H, H-14). 0.92 (d, J = 6.9 Hz, 3H, H-15). 13C NMR (125 MHz, CDCl3) δ 198.2 (C-8, C), 166.2 (C-10, C), 143.6 (C-11, C), 127.0 (C-9, CH), 114.2 (C-13, CH2), 66.8 (C-2, CH), 51.0 (C-7, CH), 41.2 (C-6, CH2), 40.0 (C-1, CH2), 39.3 (C-5, C), 37.1 (C-3, CH2), 36.3 (C-4, CH), 20.0 (C-12, CH3), 15.4 (C-14, CH3), 14.7 (C-15, CH3).
Warburgiadione (10): purified through analytical HPLC (n-hexane:acetone 95:5, flow 1.0 mL/min, tR = 32 min. 1H NMR (500 MHz, CDCl3) δ 6.99 (dt, J = 9.8, 0.7 Hz, 1H, H-1), 6.21 (dd, J = 9.8, 0.7 Hz, 1H, H-2), 6.07 (t, J = 0.7 Hz, 1H, H-9), 2.99 (d, J = 13.9 Hz, 1H, H-6β), 2.61 (q, J = 6.8 Hz, 1H, H-4), 2.38 (br d, J = 13.9 Hz, 1H, H-6α), 2.18 (d, J = 2.2 Hz, 3H, H-12)l, 1.92 (d, J = 1.5 Hz, 3H, H-13)l, 1.17 (d, J = 6.8 Hz, 3H, H-15), 0.98 (s, 3H, H-14). 13C NMR (125 MHz, CDCl3) δ 200.0 (C-3, C), 190.6 (C-8, C), 156.6 (C-10, C), 148.4 (C-11, C), 142.0 (C-1, CH), 131.8 (C-2, CH), 131.6 (C-9, CH), 126.3 (C-7, C), 51.4 (C-4, CH), 41.4 (C-5, C), 40.0 (C-6, CH2), 23.4 (C-12, CH3)m, 23.0 (C-13, CH3)m, 18.1 (C-14, CH3), 7.1 (C-15, CH3). l,m Interchangeable assignments.
Purification of the extracts fermented in PDB medium for 14 days (331 mg) and 28 days (261 mg) only afforded tyrosol (8 mg and 18.0 mg for 14 and 28 days, respectively) and 2-phenylethanol (88 mg for 14 days and 80.0 mg for 28 days).
2.4.2 Shaken culture fermentation
The resulting extract (388 mg) of the Czapek Dox fermentation was chromatographed and subsequently purified employing HPLC to yield the known compounds 4 (2.8 mg), 5 (4.0 mg), 6 (4.0 mg), 7 (0.6 mg), 9 (10.0 mg), 10 (2.0 mg), 11 (1.2 mg), 12 (1.6 mg), 13 (6.0 mg), 14 (0.5 mg), and 15 (9.2 mg) (Figure 1).
Chromatography and purification of the extract fermented in PDB medium (168 mg) yielded the known compounds 4 (8.0 mg), 9 (0.2 mg), 16 (0.5 mg), and 17 (1.0 mg) (Figure 1).
(1R,7S,8aR)-1,8a-Dimethyl-7-(prop-1-en-2-yl)-1,7,8,8a-tetrahydronaphthalene-2,6-dione (11): purified through analytical HPLC (n-hexane:acetone 95:5, flow 1.0 mL/min, tR = 39 min). +117° (c 0.38, CHCl3). 1H NMR (500 MHz, CDCl3) δ 7.03 (d, J = 9.8 Hz, 1H, H-1), 6.26 (d, J = 9.8 Hz, 1H, H-2), 6.09 (s, 1H, H-9), 5.07 (p, J = 1.5 Hz, 1H, H-13), 4.91 (dt, J = 1.5, 0.8 Hz, 1H, H-13’), 3.24 (dd, J = 13.5, 5.0 Hz, 1H, H-7), 2.63 (q, J = 6.8 Hz, 1H, H-4), 2.16 (d, J = 13.5 Hz, 1H, H-6), 2.09 (dd, J = 13.5, 5.0 Hz, 1H, H-6’), 1.78 (d, J = 1.5, 3H, H-12), 1.25 (s, 3H, H-14), 1.18 (d, J = 6.8 Hz, 3H, H-15). 13C NMR (125 MHz, CDCl3) δ 199.7 (C-3, C), 198.3 (C-8, C), 158.7 (C-10, C), 142.6 (C-11, C), 142.0 (C-1, CH), 132.2 (C-2, CH), 129.0 (C-9, CH), 115.3 (C-13, CH2), 52.2 (C-4, CH), 50.9 (C-7, CH), 40.4 (C-5, C), 40.3 (C-6, CH2), 19.9 (C-12, CH3), 18.6 (C-14, CH3), 7.0 (C-15, CH3).
Eremofortine B (12): purified through analytical HPLC (n-hexane: EtOAc 55:45, flow 1.0 mL/min, tR = 22 min). +115° (c 0.09, CHCl3). 1H NMR (400 MHz, CDCl3) δ 6.20 (s, 1H, H-9), 5.00 (br s, 1H, H-13), 4.84 (br s, 1H, H-13’), 4.12 (dt, J = 9.7, 5.0 Hz, 1H, H-3), 3.85 (dd, J = 5.0, 3.7 Hz, 1H, H-2), 3.69 (d, J = 3.7 Hz, 1H, H-1), 3.26 (dd, J = 14.1, 4.6 Hz, 1H, H-7), 1.90 (dd, J = 12.9, 4.6 Hz, 1H, H-6), 1.83 – 1.74 (m, 1H, H-6’), 1.72 (s, 3H, H-12), 1.51 (m, 1H, H-4), 1.35 (s, 3H, H-14), 1.09 (d, J = 7.1 Hz, 3H, H-15).
3β-hydroxy-7β-eremophil-1(2),9(10),11(12)-trien-8-one (13): purified through analytical HPLC (n-hexane:EtOAc 70:30, flow 1.0 mL/min, tR = 22 min). +377° (c 0.25, CHCl3). 1H NMR (400 MHz, CDCl3) δ 6.31 – 6.23 (m, 2H, H-1, H-2), 5.79 (s, 1H, H-9), 4.98 (p, J = 1.5 Hz, 1H, H-13), 4.86 (dt, J = 1.8, 0.8 Hz, 1H, H-13’), 4.18 (q, J = 4.6 Hz, 1H, H-3), 3.24 (dd, J = 13.9, 4.9 Hz, 1H, H-7), 2.01 (dd, J = 12.9, 4.9 Hz, 1H, H-6)n, 1.85 (dd, J = 13.9, 12.9 Hz, 1H, H-6’)n, 1.76 (td, J = 7.2, 4.6 Hz, 1H, H-4), 1.72 (dd, J = 1.5, 0.8 Hz, 3H, H-12), 1.32 (s, 3H, H-14), 1.15 (d, J = 7.2 Hz, 3H, H-15). 13C NMR (100 MHz, CDCl3) δ 199.0 (C-8, C), 161.6 (C-10, CH), 143.7 (C-11, C), 136.1 (C-2, CH), 129.3 (C-1, CH), 125.5 (C-9, CH), 114.7 (C-13, CH3), 68.2 (C-3, CH), 51.4 (C-7, CH), 42.0 (C-4, CH), 40.4 (C-6, CH2), 36.2 (C-5, C), 19.8 (C-12, CH3), 18.9 (C-14, CH3), 10.4 (C-15, CH3). n Interchangeable assignments.
PR toxin (14): purified through analytical HPLC (n-hexane:EtOAc 60:40, flow 1.0 mL/min, tR = 29 min). +239° (c 0.10, CHCl3). 1H NMR (500 MHz, CDCl3) δ 9.70 (s, 1H, H-12), 6.41 (s, 1H, H-9), 5.13 (t, J = 5.1 Hz, 1H, H-3), 3.95 (dd, J = 5.1, 3.5 Hz, 1H, H-2), 3.63 (d, J = 3.5 Hz, 1H, H-1), 2.15 (s, 3H, H-17), 2.14 (d, J = 14.2 Hz, 1H, H-6α), 1.82 (d, J = 14.2 Hz, 1H, H-6β), 1.77 (qd, J = 7.1, 5.1 Hz, 1H, H-4), 1.47 (s, 3H, H-13), 1.43 (s, 3H, H-14), 1.01 (d, J = 7.1 Hz, 3H, H-15). 13C NMR (125 MHz, CDCl3) δ 198.6 (C-12, C), 191.6 (C-8, C), 170.6 (C-16, C), 164.5 (C-10, C), 129.9 (C-9, CH), 69.8 (C-3, CH), 67.4 (C-11, C), 67.3 (C-7, C), 55.9 (C-1, CH), 55.5 (C-2, CH), 42.8 (C-4, CH), 41.6 (C-6, CH2), 38.1 (C-5, C), 21.9 (C-14, CH3), 20.7 (C-17, CH3), 13.7 (C-13, CH3), 10.1 (C-15, CH3).
PR toxin 3-deacetyl (15): purified through analytical HPLC (n-hexane:EtOAc 60:40, flow 1.0 mL/min, tR = 9 min). -142.7° (c 0.70, CHCl3). 1H NMR (400 MHz, CDCl3) δ 9.70 (s, 1H, H-12), 6.42 (s, 1H, H-9), 4.13 (dt, J = 9.8, 5.1 Hz, 1H, H-3), 3.91 (dd, J = 5.1, 3.6 Hz, 1H, H-2), 3.76 (d, J = 3.6 Hz, 1H, H-1), 2.10 (dd, J = 14.4, 1.1 Hz, 1H, H-6α), 1.85 (d, J = 14.4 Hz, 1H, H-6β), 1.58 – 1.50 (m, 1H, H-4), 1.47 (s, 3H, H-13), 1.42 (d, J = 1.0 Hz, 3H, H-14), 1.12 (d, J = 7.1 Hz, 3H, H-15).
(3S)-Acetoxy-7(11)-epoxyeremophil-9-en-8-one (16): purified through semipreparative HPLC (n-hexane:EtOAc 60:40, flow 3.0 mL/min, tR = 42 min). +72° (c 0.51, CHCl3). 1H NMR (600 MHz, CDCl3) δ 5.94 (s, 1H, H-9), 5.12 (q, J = 3.4 Hz, 1H, H-3), 2.73 (td, J = 13.5, 4.7 Hz, 1H, H-1β), 2.20 (m, 1H, H-1α), 2.19 (ddt, J = 14.2, 4.7, 2.3 Hz, 1H, H-2β), 2.12 (s, 3H, H-17), 2.10 (d, J = 15.0 Hz, 1H, H-6)p, 2.04 (d, J = 15.0 Hz, 1H, H-6’)p, 1.89 (qd, J = 7.0, 3.4 Hz, 1H, H-4), 1.67 (tt, J = 14.2, 3.8 Hz, 1H, H-2α), 1.41 (s, 3H, H-12), 1.32 (s, 3H, H-14), 1.31 (s, 3H, H-13), 1.03 (d, J = 7.0 Hz, 3H, H-15). 13C NMR (150 MHz, CDCl3) δ 195.0 (C-8, C), 170.7 (C-10, C), 170.3 (C-16, C), 124.1 (C-9, CH), 72.5 (C-3, CH), 65.4 (C-7, C), 64.8 (C-11, C), 42.7 (C-4, CH), 41.9 (C-5, C), 38.0 (C-6, CH2), 33.9 (C-2, CH2), 28.3 (C-1, CH2), 23.8 (C-14, CH3), 21.3 (C-12, CH3), 21.3 (C-17, CH3), 19.2 (C-13, CH3), 12.2 (C-15, CH3). p Interchangeable assignments.
Eremofortine A (17): purified through semipreparative HPLC (n-hexane:EtOAc 60:40, flow 1.0 mL/min, tR = 54 min). +137° (c 0.07, CHCl3). 1H NMR (500 MHz, CDCl3) δ 6.38 (s, 1H, H-9), 5.13 (t, J = 5.0 Hz, 1H, H-3), 3.89 (dd, J = 5.0, 3.5 Hz, 1H, H-2), 3.63 (d, J = 3.5 Hz, 1H, H-1), 2.14 (s, 3H, H-17), 2.12 (d, J = 14.7 Hz, 1H, H-6α), 1.92 (d, J = 14.7 Hz, 1H, H-6β), 1.77 (m, 1H, H-4), 1.52 (s, 3H, H-13), 1.39 (s, 3H, H-12), 1.30 (s, 3H, H-14), 1.00 (d, J = 7.1 Hz, 3H, H-15). 13C NMR (125 MHz, CDCl3) δ 193.9 (C-8, C), 170.7 (C-16, C), 160.7 (C-10, C), 131.4 (C-9, CH), 69.9 (C-3, CH), 64.6 (C-11, C), 62.4 (C-7, C), 55.9 (C-1, CH), 55.1 (C-2, CH), 42.0 (C-4, CH), 41.8 (C-6, CH2), 37.2 (C-5, C), 22.9 (C-14, CH3), 21.6 (C-12, CH3), 20.7 (C-17, CH3), 19.5 (C-13, CH3), 10.4 (C-15, CH3).
2.5 Acetylation of compound 15
Compound 15 (0.97 mg, 0.0035 mmol) was treated with a small crystal of p-toluenesulfonic acid in 2 mL of acetic anhydride. The reaction was stirred for 24 h at room temperature, neutralized with H2O and Na2CO3 and extracted with ethyl acetate. The organic layer was dried over dry Na2SO4 and the solvent eliminated by distillation under reduced pressure. Finally, the product was purified using an analytical normal-phase HPLC column (LiChrospher Si 60, 250 x 4 mm, 5 µm) applying an isocratic method (n-hexane:EtOAc 70:30, 1 mL/min) to yield 14 (0.25 mg, 0.0008 mmol, 22% yield, tR 31 min).
2.6 Computational details of EDC calculations
The molecular structure analysis of compounds 1-3 employed the semiempirical PM6 method (Stewart, 2007). Quantum mechanical computations were carried out using the Gaussian 16 package (Frisch et al., 2016). A comprehensive geometric optimization was conducted with Density Functional Theory (DFT) employing B3LYP functionals (Lee et al., 1988; Becke, 1993) and the 6−311+G(2d,p) basis set. Following this, calculations were executed to determine energies, oscillator strengths, and rotational strengths for the initial 20 electronic excitations, employing the TD-DFT methodology (Bauernschmitt and Ahlrichs, 1996; Casida et al., 1998). The solvent effect (methanol) was considered in the calculations using the Polarizable Continuum Model (PCM) with the Implicit Solvation Energy (IEF) approach (Cancès et al., 1997; Mennucci et al., 1997; Tomasi et al., 2005). To replicate the ECD spectrum of the conformer, a Gaussian function was utilized with a half-bandwidth of 0.33 eV.
2.7 In vitro antimicrobial assays
The antimicrobial activities of compounds 1, 3, 11, and 15 were evaluated against six bacterial and two fungal human pathogens. Antibacterial susceptibility of the compounds was tested against Acinetobacter baumannii ATCC19606, Escherichia coli ATCC25922, Pseudomonas aeruginosa PAO-1, Klebsiella pneumoniae ATCC700603, and methicillin-resistant Staphylococcus aureus MB5393, and sensitive S. aureus ATCC29213, while antifungal activity was tested against Aspergillus fumigatus ATCC46645 and Candida albicans ATCC64124 following previously described methodologies (Zhang et al., 2013). Briefly, each compound was serially diluted in 100% DMSO with a dilution factor of 2 to provide 10 final assay concentrations in all antimicrobial assays. Rifampicin, aztreonam, vancomycin, gentamicin and amphotericin were used as positive controls, as shown in Table 2. The MIC was defined as the lowest concentration of the compound that inhibited ≥90% of the growth of a microorganism after overnight incubation. Genedata Screener software, version 18.0.4-Standard (Genedata, Inc., Basel, Switzerland) was used to process and analyze the data and to calculate the RZ’ factor, which predicts the robustness of an assay (Zhang et al., 2013). In all experiments performed in this work, the RZ’ factor obtained was between 0.89 and 0.98.
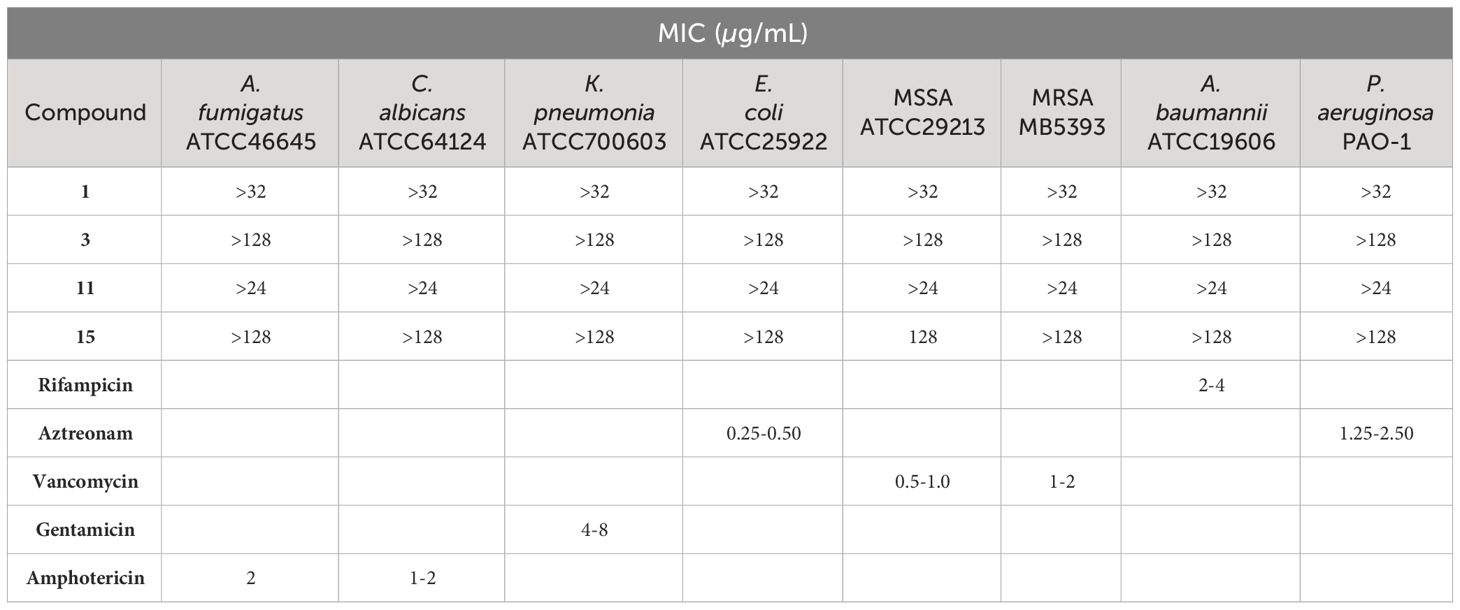
Table 2 Minimum inhibitory concentration (MIC) of compounds 1, 3, 11, and 15, and the corresponding positive controls, against eight human pathogens.
2.8 In vitro antitumoral assays
The tumor human cell lines used were liver HepG2 (ATCC HB-8065), breast MCF-7 (ATCC HTB-22), lung A549 (ATCC CCL-185), skin A2058 (ATCC CRL-3601) and pancreas Mia PaCa-2 (ATCC CRL-1420). Purified compounds were dissolved in DMSO 100% at 9 mM (compound 1), 34.4 mM (compound 3), 6.4 mM (compound 11), and at 28.8 mM (compound 15). The compounds were assayed for cytotoxicity using MTT test. The MTT test is a colorimetric assay for measuring the activity of enzymes that reduce 3-(4,5-dimethylthiazol-2-yl)-2,5-diphenyltetrazolium bromide, a yellow tetrazole, to formazan dyes, giving a purple color. Briefly, after 24 h, seeded cells were treated with compounds at a maximum dilution of 1/200 from the stocks in 10 points of 2-fold dilution per triplicate, for 72 h. MMS (Sigma Aldrich, St. Louis, Missouri, United States), at 4 mM, was used as a positive control of cell death, DMSO 0.5% as a negative control and doxorubicin (Sigma-Aldrich) was included as a known chemotherapeutic agent. After the incubation period, the plates containing treated cells were washed with 200 µL of phosphate-buffered saline (PBS) 1X (137 mM NaCl, 2.7 mM KCl, 10 mM Na2HPO4, 1.8 mM KH2PO4). Then, MTT dye (Thiazolyl blue tetrazolium bromide, ACROS Organics BV, Geel, Belgium) was added at 0.5 mg/mL and plates were incubated for 2 h. The supernatant was then removed and 100 µL of DMSO 100% were added to each well in order to dissolve the resulting formazan precipitates. Finally, absorbance was measured at 570 nm with an EnVision Multilabel Plate Reader (PerkinElmer, Waltham, USA). Data obtained was analyzed using Genedata Screener Software inhibitory curves fit to a Hill Equation model to calculate IC50 and the confidence intervals at 95%.
3 Results and discussion
The fungus E. maritima BC17 was cultivated in the liquid culture media Czapek-Dox and PDB, and incubated for 14 or 28 days in surface and shaken conditions, as described in Materials and Methods. After extraction and purification by HPLC, in addition to fourteen known compounds (4-17), three new eremophilanes (1-3) were isolated and characterized. In particular, the undescribed eremophilanes 1-3 were only isolated from Czapek-Dox fermentation broth, which showed the highest chemical diversity.
The molecular formula for compound 1 was established as C12H16O4, deduced from the sodiated molecular ion [M+Na]+ (m/z 247.0933 [M+Na]+, calculated for C12H16O4Na 247.0946) observed in its HRESIMS (Supplementary Figure S7). Its IR spectrum showed absorption bands characteristic for hydroxy (3447 cm−1) and enone carbonyl groups (1637 cm−1). The 1H NMR data (Table 1) indicated the presence of two methyl signals at δH 1.28 (d, J = 7.0 Hz) and 1.35 (s), and two olefinic signals at δH 6.17 (d, J = 1.5 Hz) and 6.30 (s). The 13C NMR data (Table 1) displayed twelve signals including one carbonyl (δC 183.9), four olefinic carbons (δC 124.4, 127.4, 147.9, 171.1), two methyls (δC 13.2, 22.0), one methylene (δC 37.5), other three methines, two of which were oxygenated (δC 43.4, 74.1, 74.6), and another quaternary carbon (δC 45.0).
Two double bonds and one carbonyl group accounted for three of five degrees of unsaturation, indicating the presence of two rings in the structure. The two-ring system was then established based on 1H−1H COSY (Supplementary Figure S3), HSQC (Supplementary Figure S4), and HMBC (Supplementary Figure S5) correlations (Figure 2). Inspection of the 1H−1H COSY and HSQC data led to the assignment of a C(1)H2−C(2)H−C(3)H−C(4)H−C(15)H3 unit. The assigned spin system, together with HMBC correlations from H2-1 to C-3/C-5/C-9/C-10, from H-4 to C-14, and from H-15 to C-3/C-4/C-5, permitted the complete elucidation of ring A. HMBC correlations from H-6 to C-7/C-8/C-10/C-4/C-14 and from H-9 to C-1/C-5/C-7 allowed the elucidation of ring B. Thus, compound 1 was established as a nor-eremophilane sesquiterpene. Its 1H and 13C NMR spectra (Supplementary Figures S1, S2) were very similar to those of compound guignarderemophilane A (18), previously reported as a metabolite from the endophytic fungus Guignardia mangiferae (Liu et al., 2015), except for the absence of the acetyl group signals at C-3.
The relative configuration of 1 was determined by analysis of 1D NOESY (Supplementary Figures S6a–g) correlations and the splitting patterns of related protons (Figure 3). The NOESY correlations between H-2, H-3 and H-4 revealed that they showed the same orientation. The NOE effect between the equatorial Me-15 and H-6 revealed that C5−C6 was equatorial and that Me-14 was axial. The correlations between Me-14 and Me-15 suggested they were in the syn conformation. These correlations permitted us to propose the relative configuration of 1 as shown in Figure 3.
Absolute stereochemistry of compound 1 was established by comparison of the experimental electronic circular dichroism (ECD) spectrum with the one obtained from quantum mechanical time-dependent density functional theory (TD-DFT) calculations for the (2S,3R,4R,5S) stereoisomer in the 200-400 nm region (Figure 4) (Li et al., 2010). Moreover, compound 1 showed an ECD spectrum similar to that of the previously reported acetylated compound guignarderemophilane A (18) (Liu et al., 2015). Based on these data, the absolute configuration at C-2, -3, -4 and -5 was determined to be S, R, R, and S, respectively. Therefore, the structure of 1 was characterized and named as 3-deacetyl guignarderemophilane A.
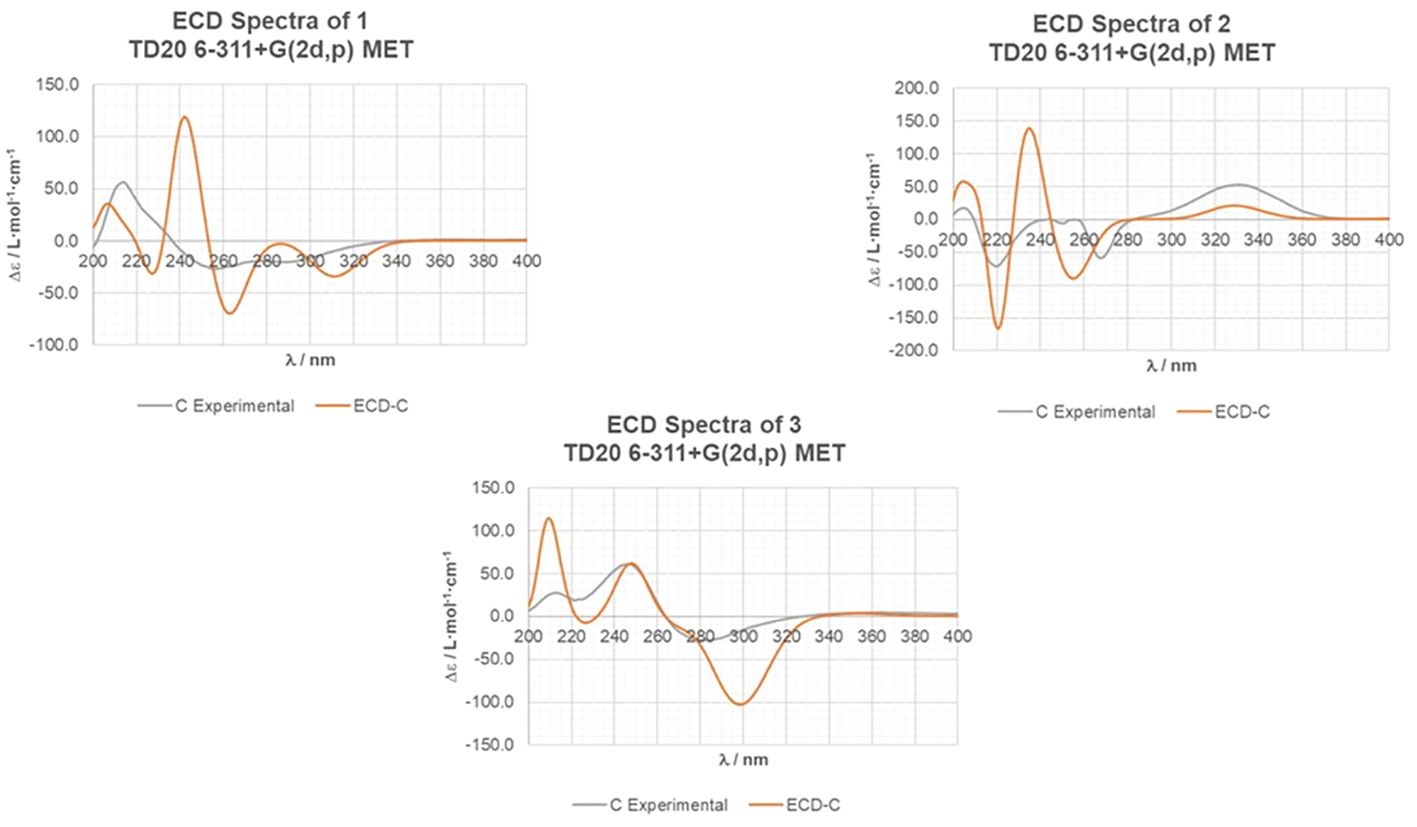
Figure 4 Experimental and calculated ECD spectra for compounds 1-3. Calculations were performed with the conformers shown in Figure 3.
Compound 2 was obtained as a colourless oil with the molecular formula C15H22O3, as determined by the HRESIMS peak at m/z 273.1446 [M+Na]+ (Supplementary Figure S14), indicating five degrees of unsaturation. Its IR spectrum showed absorptions corresponding to a hydroxyl group (3496 cm−1) and an α,β-unsaturated keto group (1674 cm−1). The 13C NMR data (Table 1) displayed fifteen carbon resonances, some of which could be assigned to one keto group (δC 195.2), a C=C double bond (δC 123.6, 172.4), one 1,2-epoxy group (δC 64.7, 65.6), one oxygenated sp3 carbon (δC 70.8), and four methyl groups in the high-field region (δC 12.6, 19.1, 21.3, 24.5). These functionalities account for three degrees of unsaturation, thus hinting the bicyclic nature of 2. Analysis of the 1H NMR data (Table 1) revealed the presence of four methyl groups, including three singlets (δH 1.30, 1.36, 1.41) and one doublet (δH 1.15, J=7.1 Hz) in the high-field region. These data were characteristic of an eremophilane-type sesquiterpene, i.e., a cis-decaline ring with an isopropyl group (C-11/Me-12/Me-13) and two methyl groups, one connected to a methine group (CH-4) and the other one connected to a quaternary carbon (C-5), resulting in one doublet (Me-15) and one singlet (Me-14) signals (Supplementary Figure S11). In addition, the signals for one olefinic proton (δH 5.92) and one oxymethine proton (δH 3.97) could be seen in the 1H NMR spectrum (Supplementary Figure S8), which in combination with the signals observed in the 13C NMR spectrum (Supplementary Figure S9) suggested the occurrence of one trisubstituted double bond, and one oxygenated methine group. The positions of these functional groups in the eremophilane skeleton were clarified by analysis of the HMBC spectrum (Supplementary Figure S12). The presence of an epoxide moiety with 13C peaks at C-7 (δC 65.6) and C-11 (δC 64.7) was confirmed by HMBC correlations from Me-13 (δH 1.30) to C-7, C-11 and C-12 (δC 21.3), from H2-6 (δH 2.08 and 1.99) to C-7, C-10 (δC 172.4), C-11, and C-14 (δC 24.5), and from Me-12 (δH 1.41) to C-7, C-11, and C-13 (δC 19.1) (Figure 2). Correlation between Me-15 (δH 1.15, d) and the oxygenated methine (δC 70.8) placed the hydroxyl substituent at C-3 (Figure 2). The 1H and 13C NMR spectra of 2 (Supplementary Figures S8, S9) were very similar to those of compound 3-acetoxy-7(11)-epoxyeremophil-9-en-8-one (16), previously reported by our research’s group as an E. maritima BC17 metabolite from solid culture media (Virués-Segovia et al., 2023), except for the absence of the acetyl group signals at C-3.
The relative configuration of 2 was determined by analysis of 1D NOESY correlations (Supplementary Figures S13a–f). The H-1 (δH 2.90) and Me-14 protons showed strong NOE correlations with each other, indicating that H-1β and Me-14 were in axial orientations on the same face of ring A. The NOE correlations between H-3 and H-1 (δH 2.16) and H-4 confirmed that these protons were on the opposite side from H-1β/Me-14. NOE correlations between Me-14 and Me-15 indicated both methyls were on the same face. The β-orientation of the epoxide at C-7 and C-11 in 2 was evidenced by the NOE effect from Me-12 to H-6α (δH 2.08) (Figure 3).
The ECD spectrum of 2 was very similar to that obtained for the previously reported compound 16 (Virués-Segovia et al., 2023). In addition, the ECD curve for the (3S,4R,5R,7R) stereoisomer, calculated with the TD-DFT theoretical method, matched well with the experimental ECD spectrum of 2 (Figure 4). As a result, its absolute stereochemistry was assigned as (3S,4R,5R,7R)-3-hydroxy-7(11)-epoxyeremophil-9-en-8-one (2).
Compound 3 had a molecular formula of C15H20O2, as deduced from HRESIMS (Supplementary Figure S21), which was consistent with six degrees of unsaturation. The 13C NMR data (Table 1) displayed fifteen signals, including two carbonyl groups (δC 190.5, 212.1), four olefinic carbons (δC 127.0, 128.2, 146.1, 162.6), four methyls (δC 11.0, 22.8, 23.1, 25.1), three methylenes (δC 29.0, 37.4, 37.5), another methine (δC 53.6), and an additional quaternary carbon (δC 42.2). The 1H NMR data (Table 1) showed four methyl groups, including one singlet (δH 1.20) and three doublets (δH 1.10, J=7.0 Hz; 1.85, J=1.4 Hz; 2.15, J=2.0 Hz) in the high-field region.
NMR data (Table 1) of 3 revealed a great similarity with those of isopetasone (4) (Brooks and Draffan, 1969; Yamakawa et al., 1974). However, the 1H and 13C NMR spectra (Supplementary Figures S15, S16) of both compounds differed significantly in the shift of the signals corresponding to H-1β (δH 2.87 in 3 and 2.72 in 4), H-6α (δH 2.49 in 3 and 2.82 in 4), Me-14 (δC/H 25.1/1.20 in 3 and 18.5/0.96 in 4), and Me-15 (δC 11.0 in 3 and 7.4 in 4). This suggested a possible difference in the stereochemistry of these methyls. The NOESY correlation between H-1 (δH 2.87) and Me-14 (δH 1.20) (Supplementary Figures S20a, d) revealed that this methyl group was axial, while the correlation from Me-14 to H-4 (δH 2.60) (Supplementary Figure S20d) positioned the latter to the equatorial site, indicating that Me-14 and H-4 shared the same β-configuration (Figure 3), in contrast to all eremophilanes described in this work. Hence, compound 3 was elucidated to be 4-epiisopetasone.
This stereochemistry was confirmed by comparison of the predicted ECD spectrum from TD-DFT calculations with the experimental one of compound 3. The calculated spectrum reproduced well the experimental data (Figure 4), confirming the 4S and 5R absolute configuration of 3.
In view of these results, it is noteworthy that the new metabolites could only be isolated under surface culture conditions and with Czapek-Dox medium. In particular, compound 3 belongs to a different stereochemical series than all eremophilanes previously isolated from the E. maritima BC17 strain. This indicates that the conditional changes are useful for accessing cryptic metabolites that would not be obtained under standard laboratory conditions.
The other isolated compounds 4-17 were identified by comparing their spectroscopic data with the literature: isopetasone (4) (Brooks and Draffan, 1969; Yamakawa et al., 1974) (Supplementary Figures S22, S23), (+)-3-epiisopetasol (5) (Sumarah et al., 2010) (Supplementary Figures S24, S25), 1α-hydroxydehydrofukinone (6) (Bohlmann and Knoll, 1979) (Supplementary Figures S26, S27), eremofortin A alcohol (7) (Moreau et al., 1977) (Supplementary Figures S28, S29), (+)-aristolochene (8) (Demyttenaere et al., 2002) (Supplementary Figures S30, S31), 7-hydroxy-4a,5-dimethyl-3-prop-1-en-2-yl-3,4,5,6,7,8-hexahydronaphthalen-2-one (9) (Daengrot et al., 2015) (Supplementary Figures S32, S33), warburgiadione (10) (Brooks and Draffan, 1969) (Supplementary Figures S34, S35), (1R,7S,8aR)-1,8a-dimethyl-7-(prop-1-en-2-yl)-1,7,8,8a-tetrahydronaphthalene-2,6-dione (11) (Fan, 2016) (Supplementary Figures S36, S37), eremofortine B (12) (Moreau et al., 1980) (Supplementary Figure S38), 3β-hydroxy-7β-eremophil-1(2),9(10),11(12)-trien-8-one (13) (Lin et al., 2014) (Supplementary Figure S39, S40), PR toxin (14) (Moreau et al., 1980) (Supplementary Figures S41, S42), PR toxin 3-deacetyl (15) (Wei et al., 1975; Capasso et al., 1986) (Supplementary Figure S43), (3S)-acetoxy-7(11)-epoxyeremophil-9-en-8-one (16) (Virués-Segovia et al., 2023) (Supplementary Figures S44, S45), and eremofortine A (17) (Moreau et al., 1980) (Supplementary Figures S46, S47). Compounds 11, 12, and 15 are reported for the first time as fungal metabolites from E. maritima BC17 in this work, indicating the usefulness of the OSMAC strategy to increase the chemical diversity of the strain under study.
PR toxin 3-deacetyl (15) was previously chemically synthesised from PR toxin (14) (Wei et al., 1975). In order to confirm the configuration at C-3 of 15, this compound was acetylated with acetic anhydride and p-toluenesulfonic acid to give a product whose spectroscopic data coincided with those found in literature for PR toxin (14) (Moreau et al., 1980). This has allowed us to confirm the absolute configuration of compound 15 and report it for the first time as a fungal metabolite.
The undescribed isolated metabolites 1 and 3 and the known compounds 11 and 15 were evaluated for antimicrobial and cytotoxic activities. They were tested in triplicates against a panel of 8 human pathogens, including both Gram-negative and Gram-positive bacteria (E. coli ATCC25922, P. aeruginosa PAO-1, A. baumannii ATCC19606, K. pneumoniae ATCC700603, methicillin-resistant S. aureus MB5393, and sensitive S. aureus ATCC29213), yeast (C. albicans ATCC64124) and fungal (A. fumigatus ATCC46645) strains. No antimicrobial activity was detected for these metabolites against the human pathogenic strains assayed, except for compound 15 that exhibited selective but moderate activity against methicillin-sensitive S. aureus ATCC29213 at the highest concentration tested of 128 µg/mL (Table 2).
Antitumoral activity of these compounds (1, 3, 11, and 15) was also tested against liver (HepG2, ATCC HB-8065), breast (MCF-7, ATCC HTB-22), lung (A549, ATCC CCL-185), skin (A2058, ATCC CRL-3601), and pancreas (Mia PaCa-2, ATCC CRL-1420) human cancer cells using MTT test. Methyl methanesulfonate (MMS) 4 mM was used as positive control of cell death and doxorubicin was included as known chemotherapeutic agent.
In previous studies, in vitro tests of compound 11, isolated from dry root of Valeriana jatamansi, indicated that it played a restraining effect to human brain malignant glioblastoma U251 cell by inhibiting cell proliferation and inducing cell apoptosis (Fan, 2016). However, in this work we did not detect antiproliferative activity of this metabolite, nor of compounds 1 and 3, against the human cancer cells assayed. Among the eremophilanes tested, only compound 15 was active against all of the tested cell lines with IC50 values ranging from 2.5 to 14.7 µM (Table 3). In a previous study, we reported that the PR toxin (14) inhibited the same human cancer cells tested with IC50 values in the range of 3.75-33.44 µg/mL (Virués-Segovia et al., 2023). These results confirm that the aldehyde group at C-12 present in both compounds, 14 and 15, is directly related to their biological activity.
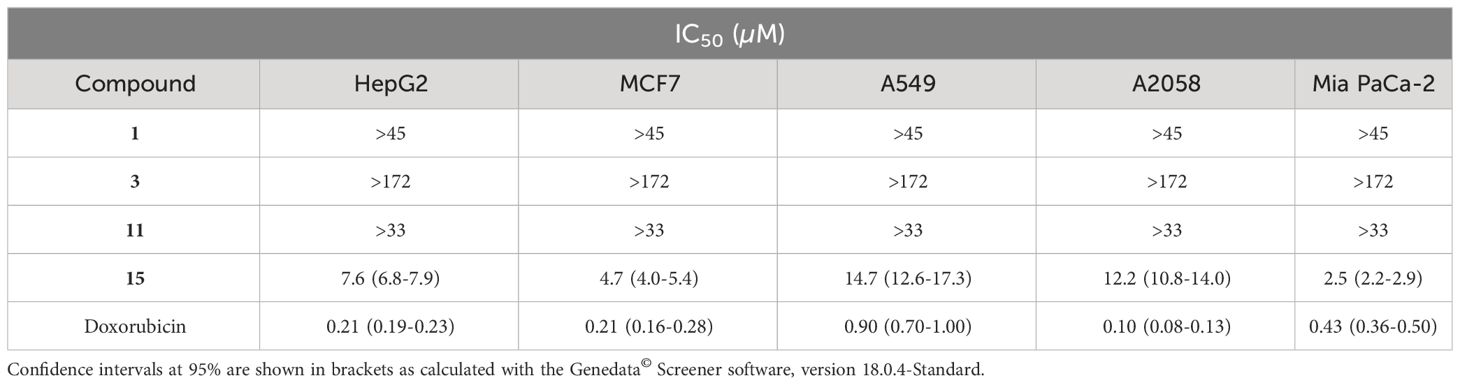
Table 3 Half-maximal inhibitory concentration (IC50) of compounds 1, 3, 11, and 15 of cell viability in five tumor cell lines.
4 Conclusions
In this research, three previously undescribed eremophilanes (1-3) and fourteen known eremophilane derivatives (4-17) were isolated and identified from the fungal strain E. maritima BC17, grown in both Czapek Dox and PDB liquid culture media, in order to increase its chemical diversity. Notably, the new eremophilanes 1-3 were only isolated from the Czapek-Dox medium, which showed the highest chemical diversity. Compound 3 belongs to a different stereochemical series than all eremophilanes previously isolated from the strain under study (Virués-Segovia et al., 2023). These results confirm that the OSMAC approach is a simple, rapid and effective strategy to increase the chemical diversity of marine natural products by activating silent gene clusters.
In addition, compounds 1, 3, 11 and 15 were tested for antimicrobial and cytotoxic activity. Compound 15 exhibited cytotoxic activity against HepG2, MCF-7, A549, A2058 and Mia PaCa-2 human cancer cell lines with IC50 values ranging from 2.5 to 14.7 µM. The aldehyde group at C-12 was identified as responsible for its biological activity, as observed in compound 14 (Virués-Segovia et al., 2023).
Data availability statement
The original contributions presented in the study are included in the article/Supplementary Material. Further inquiries can be directed to the corresponding authors.
Ethics statement
Ethical approval was not required for the studies on humans in accordance with the local legislation and institutional requirements because only commercially available established cell lines were used.
Author contributions
JRV-S: Writing – review & editing, Investigation. CP: Writing – review & editing, Methodology, Investigation. DZ: Writing – review & editing, Data curation. JS-M: Writing – review & editing, Data curation. PS: Writing – review & editing, Data curation. MCR: Writing – review & editing, Data curation. MdlC: Writing – review & editing, Data curation. JA: Writing – review & editing, Writing – original draft, Supervision, Project administration, Funding acquisition, Conceptualization. RD-P: Writing – review & editing, Supervision, Methodology, Funding acquisition, Conceptualization.
Funding
The author(s) declare financial support was received for the research, authorship, and/or publication of this article. This work was co-financed by the 2014-2020 ERDF Operational Programme and by the Department of Economy, Knowledge, Business and University of the Regional Government of Andalusia. Project reference: FEDER-UCA18-105749.
Acknowledgments
J.R. V-S thanks the Spanish Ministry of Universities for a grant of the National Programme FPU 2022.
Conflict of interest
The authors declare that the research was conducted in the absence of any commercial or financial relationships that could be construed as a potential conflict of interest.
Publisher’s note
All claims expressed in this article are solely those of the authors and do not necessarily represent those of their affiliated organizations, or those of the publisher, the editors and the reviewers. Any product that may be evaluated in this article, or claim that may be made by its manufacturer, is not guaranteed or endorsed by the publisher.
Supplementary material
The Supplementary Material for this article can be found online at: https://www.frontiersin.org/articles/10.3389/fmars.2024.1386175/full#supplementary-material
References
Agrawal S., Dufossé L., Deshmukh S. K. (2023). Antibacterial metabolites from an unexplored strain of marine fungi Emericellopsis minima and determination of the probable mode of action against Staphylococcus aureus and methicillin-resistant S. aureus. Biotechnol. Appl. Biochem. 70, 120–129. doi: 10.1002/bab.2334
Agrawal S., Saha S. (2022). The genus Simplicillium and Emericellopsis: A review of phytochemistry and pharmacology. Biotechnol. Appl. Biochem. 69, 2229–2239. doi: 10.1002/bab.2281
Batista-García R. A., Kumar V. V., Ariste A., Tovar-Herrera O. E., Savary O., Peidro-Guzmán H., et al. (2017). Simple screening protocol for identification of potential mycoremediation tools for the elimination of polycyclic aromatic hydrocarbons and phenols from hyperalkalophile industrial effluents. J. Environ. Manage. 198, 1–11. doi: 10.1016/j.jenvman.2017.05.010
Bauernschmitt R., Ahlrichs R. (1996). Treatment of electronic excitations within the adiabatic approximation of time dependent density functional theory. Chem. Phys. Lett. 256, 454–464. doi: 10.1016/0009-2614(96)00440-X
Becke A. D. (1993). Density-functional thermochemistry. III. The role of exact exchange. J. Chem. Phys. 98, 5648–5652. doi: 10.1063/1.464913
Blayney D. W., Mohanlal R., Adamchuk H., Kirtbaya D. V., Chen M., Du L., et al. (2022). Efficacy of Plinabulin vs Pegfilgrastim for prevention of Docetaxel-induced neutropenia in patients with solid tumors: A randomized clinical trial. JAMA Netw. Open 5, 1–12. doi: 10.1001/jamanetworkopen.2021.45446
Blayney D. W., Zhang Q., Feng J., Zhao Y., Bondarenko I., Vynnychenko I., et al. (2020). Efficacy of Plinabulin vs Pegfilgrastim for prevention of chemotherapy-induced neutropenia in adults with non–small cell lung cancer. JAMA Oncol. 6, e204429. doi: 10.1001/jamaoncol.2020.4429
Bode H. B., Bethe B., Höfs R., Zeeck A. (2002). Big effects from small changes: Possible ways to explore nature’s chemical diversity. ChemBioChem 3, 619. doi: 10.1002/1439-7633(20020703)3:7<619::AID-CBIC619>3.0.CO;2-9
Bohlmann F., Knoll K. (1979). Natürlich vorkommende terpen-derivate, 200 zwei neue eremophilan-derivate aus Senecio suaveolens. Liebigs Ann. der Chemie 1979, 470–472. doi: 10.1002/jlac.197919790407
Bovio E., Garzoli L., Poli A., Prigione V., Firsova D., McCormack G. P., et al. (2018). The culturable mycobiota associated with three Atlantic sponges, including two new species: Thelebolus balaustiformis and T. spongiae. Fungal Syst. Evol. 1, 141–167. doi: 10.3114/fuse.2018.01.07
Brooks C. J. W., Draffan G. H. (1969). Sesquiterpenoids of Warburgia species—I: Warburgin and warburgiadione. Tetrahedron 25, 2865–2885. doi: 10.1016/0040-4020(69)80030-X
Cancès E., Mennucci B., Tomasi J. (1997). A new integral equation formalism for the polarizable continuum model: Theoretical background and applications to isotropic and anisotropic dielectrics. J. Chem. Phys. 107, 3032–3041. doi: 10.1063/1.474659
Capasso R., Borrelli V., Iacobellis N. S., Basile G. (1986). Preparation of derivatives of phomenone and PR toxin to study the correlation between structure and biological activity of eremophilanic sesquiterpenes Vol. 20 (Naples, Italy: Ann. della Fac. di Sci. Agrar. della Univ. degli Stud. di Napoli, Portici, IV), 20–28. Available at: https://eurekamag.com/research/001/660/001660838.php.
Casida M. E., Jamorski C., Casida K. C., Salahub D. R. (1998). Molecular excitation energies to high-lying bound states from time-dependent density-functional response theory: Characterization and correction of the time-dependent local density approximation ionization threshold. J. Chem. Phys. 108, 4439–4449. doi: 10.1063/1.475855
Christaki E., Marcou M., Tofarides A. (2020). Antimicrobial resistance in bacteria: Mechanisms, evolution, and persistence. J. Mol. Evol. 88, 26–40. doi: 10.1007/s00239-019-09914-3
Daengrot C., Rukachaisirikul V., Tansakul C., Thongpanchang T., Phongpaichit S., Bowornwiriyapan K., et al. (2015). Eremophilane sesquiterpenes and diphenyl thioethers from the soil fungus Penicillium copticola PSU-RSPG138. J. Nat. Prod. 78, 615–622. doi: 10.1021/np5005328
Demyttenaere J. C. R., Adams A., Van Belleghem K., De Kimpe N., König W. A., Tkachev A. V. (2002). De novo production of (+)-aristolochene by sporulated surface cultures of Penicillium roqueforti. Phytochemistry 59, 597–602. doi: 10.1016/S0031-9422(02)00002-X
Fan S. (2016). Eremophilane type sesquiterpenoids and medical application for treating glioma (China). Pat. n° CN105503556 A 2016-04-20.
Frisch M. J., Trucks G. W., Schlegel H. B., Scuseria G. E., Robb M. A., Cheeseman J. R., et al. (2016). Gaussian 16, Revision.01; Wallingford, CT, USA: Gaussian Inc.
Gonçalves M. F. M., Vicente T. F. L., Esteves A. C., Alves A. (2020). Novel halotolerant species of Emericellopsis and Parasarocladium associated with macroalgae in an estuarine environment. Mycologia 112, 154–171. doi: 10.1080/00275514.2019.1677448
Grossart H., Van den Wyngaert S., Kagami M., Wurzbacher C., Cunliffe M., Rojas-Jiménez K. (2019). Fungi in aquatic ecosystems. Nat. Rev. Microbiol. 17, 339–354. doi: 10.1038/s41579-019-0175-8
Grum-Grzhimaylo A. A., Georgieva M. L., Debets A. J. M., Bilanenko E. N. (2013). Are alkalitolerant fungi of the Emericellopsis lineage (Bionectriaceae) of marine origin? IMA Fungus 4, 213–228. doi: 10.5598/imafungus.2013.04.02.07
Hafez Ghoran S., Taktaz F., Ayatollahi S. A., Kijjoa A. (2022). Anthraquinones and their analogues from marine-derived fungi: Chemistry and biological activities. Mar. Drugs 20, 474. doi: 10.3390/md20080474
Inostroza A., Lara L., Paz C., Perez A., Galleguillos F., Hernandez V., et al. (2018). Antibiotic activity of emerimicin IV isolated from Emericellopsis minima from Talcahuano Bay, Chile. Nat. Prod. Res. 32, 1361–1364. doi: 10.1080/14786419.2017.1344655
Jin L., Quan C., Hou X., Fan S. (2016). Potential pharmacological resources: Natural bioactive compounds from marine-derived fungi. Mar. Drugs 14, 76. doi: 10.3390/md14040076
Khalifa S. A. M., Elias N., Farag M. A., Chen L., Saeed A., Hegazy M.-E. F., et al. (2019). Marine natural products: A source of novel anticancer drugs. Mar. Drugs 17, 491. doi: 10.3390/md17090491
Kuvarina A. E., Gavryushina I. A., Kulko A. B., Ivanov I. A., Rogozhin E. A., Georgieva M. L., et al. (2021). The emericellipsins A-E from an alkalophilic fungus Emericellopsis alkalina show potent activity against multidrug-resistant pathogenic fungi. J. Fungi 7, 153. doi: 10.3390/jof7020153
Lee C., Yang W., Parr R. G. (1988). Development of the Colle-Salvetti correlation-energy formula into a functional of the electron density. Phys. Rev. B 37, 785–789. doi: 10.1103/PhysRevB.37.785
Li X.-C., Ferreira D., Ding Y. (2010). Determination of absolute configuration of natural products: Theoretical calculation of electronic circular dichroism as a tool. Curr. Org. Chem. 14, 1678–1697. doi: 10.2174/138527210792927717
Lin A., Wu G., Gu Q., Zhu T., Li D. (2014). New eremophilane-type sesquiterpenes from an Antarctic deep-sea derived fungus, Penicillium sp. PR19 N-1. Arch. Pharm. Res. 37, 839–844. doi: 10.1007/s12272-013-0246-8
Liu Y., Li Y., Qu J., Ma S., Zang C., Zhang Y., et al. (2015). Eremophilane sesquiterpenes and polyketones produced by an endophytic Guignardia fungus from the toxic plant Gelsemium elegans. J. Nat. Prod. 78, 2149–2154. doi: 10.1021/np5009027
Mennucci B., Cancès E., Tomasi J. (1997). Evaluation of solvent effects in isotropic and anisotropic dielectrics and in ionic solutions with a unified integral equation method: Theoretical bases, computational implementation, and numerical applications. J. Phys. Chem. B 101, 10506–10517. doi: 10.1021/jp971959k
Moreau S., Biguet J., Lablache-Combier A., Baert F., Foulon M., Delfosse C. (1980). Structures et stereochimie des sesquiterpenes de Penicillium roqueforti PR toxine et eremofortines A, B, C, D, E. Tetrahedron 36, 2989–2997. doi: 10.1016/0040-4020(80)88024-0
Moreau S., Cacan M., Lablache-Combier A. (1977). Eremofortin C, a new metabolite obtained from Penicillium roqueforti cultures and from biotransformation of PR toxin. J. Org. Chem. 42, 2632–2634. doi: 10.1021/jo00435a023
Pang K.-L., Overy D. P., Jones E. B. G., Calado M., da L., Burgaud G., et al. (2016). ‘Marine fungi’ and ‘marine-derived fungi’ in natural product chemistry research: Toward a new consensual definition. Fungal Biol. Rev. 30, 163–175. doi: 10.1016/j.fbr.2016.08.001
Pinedo-Rivilla C., Aleu J., Durán-Patrón R. (2022). Cryptic metabolites from marine-derived microorganisms using OSMAC and epigenetic approaches. Mar. Drugs 20, 84. doi: 10.3390/md20020084
Rogozhin E., Sadykova V. (2019). A lipoaminopeptaibol secreted by alkalophilic fungus Emericellopsis alkalina demonstrates a strong cytotoxic effect against tumor cell lines. Proceedings 22, 4. doi: 10.3390/proceedings2019022004
Song Y., Wang J., Huang H., Ma L., Wang J., Gu Y., et al. (2012). Four eremophilane sesquiterpenes from the mangrove endophytic fungus Xylaria sp. BL321. Mar. Drugs 10, 340-348. doi: 10.3390/md10020340
Stewart J. J. P. (2007). Optimization of parameters for semiempirical methods V: Modification of NDDO approximations and application to 70 elements. J. Mol. Model. 13, 1173–1213. doi: 10.1007/s00894-007-0233-4
Sumarah M. W., Puniani E., Sørensen D., Blackwell B. A., Miller J. D. (2010). Secondary metabolites from anti-insect extracts of endophytic fungi isolated from Picea rubens. Phytochemistry 71, 760–765. doi: 10.1016/j.phytochem.2010.01.015
Sung H., Ferlay J., Siegel R. L., Laversanne M., Soerjomataram I., Jemal A., et al. (2021). Global cancer statistics 2020: GLOBOCAN Estimates of incidence and mortality worldwide for 36 cancers in 185 countries. CA. Cancer J. Clin. 71, 209–249. doi: 10.3322/caac.21660
Tomasi J., Mennucci B., Cammi R. (2005). Quantum mechanical continuum solvation models. Chem. Rev. 105, 2999–3093. doi: 10.1021/cr9904009
Virués-Segovia J. R., Millán C., Pinedo C., González-Rodríguez V. E., Papaspyrou S., Zorrilla D., et al. (2023). New eremophilane-type sesquiterpenes from the marine sediment-derived fungus Emericellopsis maritima BC17 and their cytotoxic and antimicrobial activities. Mar. Drugs 21, 634. doi: 10.3390/md21120634
Wang L., Li M., Tang J., Li X. (2016). Eremophilane sesquiterpenes from a deep marine-derived fungus, Aspergillus sp. SCSIOW2, cultivated in the presence of epigenetic modifying agents. Molecules 21, 473. doi: 10.3390/molecules21040473
Wang H.-N., Sun S.-S., Liu M.-Z., Yan M.-C., Liu Y.-F., Zhu Z., et al. (2022). Natural bioactive compounds from marine fungi, (2017–2020). J. Asian Nat. Prod. Res. 24, 203–230. doi: 10.1080/10286020.2021.1947254
Wei R., Schnoes H. K., Hart P. A., Strong F. M. (1975). The structure of PR toxin, a mycotoxin from Penicillium roqueforti. Tetrahedron 31, 109–114. doi: 10.1016/0040-4020(75)85003-4
Wiese J., Ohlendorf B., Blümel M., Schmaljohann R., Imhoff J. F. (2011). Phylogenetic identification of fungi isolated from the marine sponge Tethya aurantium and identification of their secondary metabolites. Mar. Drugs 9, 561–585. doi: 10.3390/md9040561
Wu G., Lin A., Gu Q., Zhu T., Li D. (2013). Four new chloro-eremophilane sesquiterpenes from an Antarctic deep-sea derived fungus, Penicillium sp. PR19N-1. Mar. Drugs 11, 1399–1408. doi: 10.3390/md11041399
Xu L., Meng W., Cao C., Wang J., Shan W., Wang Q. (2015). Antibacterial and antifungal compounds from marine fungi. Mar. Drugs 13, 3479–3513. doi: 10.3390/md13063479
Yamakawa K., Izuta I., Oka H., Sakaguchi R. (1974). Total synthesis of (±)-isopetasol, (±)-3-epiisopetasol, and (±)-warburgiadion. Tetrahedron Lett. 15, 2187–2190. doi: 10.1016/S0040-4039(01)93172-7
Zhang J., Liu D., Fan A., Huang J., Lin W. (2022). Eremophilane-type sesquiterpenes from a marine-derived fungus Penicillium copticola with antitumor and neuroprotective activities. Mar. Drugs 20, 712. doi: 10.3390/md20110712
Zhang L., Ravipati A. S., Koyyalamudi S. R., Jeong S. C., Reddy N., Bartlett J., et al. (2013). Anti-fungal and anti-bacterial activities of ethanol extracts of selected traditional Chinese medicinal herbs. Asian Pac. J. Trop. Med. 6, 673–681. doi: 10.1016/S1995-7645(13)60117-0
Keywords: eremophilane, Emericellopsis maritima, marine-derived fungus, OSMAC approach, antimicrobial, antitumoral
Citation: Virués-Segovia JR, Pinedo C, Zorrilla D, Sánchez-Márquez J, Sánchez P, Ramos MC, de la Cruz M, Aleu J and Durán-Patrón R (2024) Discovery of new eremophilanes from the marine-derived fungus Emericellopsis maritima BC17 by culture conditions changes: evaluation of cytotoxic and antimicrobial activities. Front. Mar. Sci. 11:1386175. doi: 10.3389/fmars.2024.1386175
Received: 14 February 2024; Accepted: 22 April 2024;
Published: 10 May 2024.
Edited by:
Susana P. Gaudêncio, New University of Lisbon, PortugalReviewed by:
Hui Cui, Guangzhou University of Chinese Medicine, ChinaChang-Wei Li, Beijing Institute of Pharmacology & Toxicology, China
Copyright © 2024 Virués-Segovia, Pinedo, Zorrilla, Sánchez-Márquez, Sánchez, Ramos, de la Cruz, Aleu and Durán-Patrón. This is an open-access article distributed under the terms of the Creative Commons Attribution License (CC BY). The use, distribution or reproduction in other forums is permitted, provided the original author(s) and the copyright owner(s) are credited and that the original publication in this journal is cited, in accordance with accepted academic practice. No use, distribution or reproduction is permitted which does not comply with these terms.
*Correspondence: Josefina Aleu, josefina.aleu@uca.es; Rosa Durán-Patrón, rosa.duran@uca.es